1 Introduction
The decomposition rates of crop residues in soils are frequently considered to be regulated by interactions between soil microorganisms and their extracellular enzymes, environmental factors and plant residue quality [1–3]. The kinetics of residue C mineralization, in the short-term, have been shown to mostly depend on the amount of C present in soluble fraction of the residue [4–6]. As decomposition proceeds, the soluble components are degraded and their effect on the rate of C mineralization is reduced. Subsequent decomposition is driven by the more recalcitrant fraction, i.e. cell walls [7]. The principal cell wall polymers are polysaccharides (such as cellulose and hemicelluloses) and lignin. The main types of hemicellulose encountered in Gramineae are heteroxylans, which consist of a backbone of β-1,4-linked xylose units mainly substituted by α-linked arabinose. Previous studies have shown that the decomposition of cell walls in soil is influenced by their chemical composition [7,8] with polysaccharides (cellulose and heteroxylan) being the most readily degraded polymers, along with the less condensed and thus more accessible lignin fraction [9].
During the decomposition process, soil microorganisms are colonizing dead plant material after entering the soil [10,11]. However, plant residues also contain bacterial and fungal populations, before incorporation into soil, which might influence their decomposition dynamics [12,13]. In a recent study [13], maize roots with a high N content were shown to be more colonized than roots with a lower N content. The authors suggested that residue-colonizing microorganisms would already decompose part of the cell wall polysaccharides before soil decomposition, thus modifying the chemical quality of the residues and their subsequent mineralization patterns in soil. However, gamma (γ) sterilized and non-sterilized root residues were found to exhibit similar C mineralization rates, indicating that residue-colonizing microorganisms did not quantitatively affect soil microorganism activity. To our knowledge this type of study has not been carried out yet on residues of above-ground plant organs.
Numerous microbial communities are present on the above-ground (leaf and stem) and underground parts (root) of plants. The above-ground parts are normally colonized by a variety of fungi and bacteria that colonize the phyllosphere and are called epiphytes [14,15]. In the case of roots, rhizosphere fungi which adhere to the root epidermis and outer cortex, are primarily involved in the degradation of complex organic compounds [16,17]. While fungi and bacteria that live within the cells of the root are not considered a part of rhizosphere, but instead are called endophytes [18]. The effects of plant microorganisms or enzymes (whether associated or possibly derived from plant vestige metabolism) on decomposition are generally assessed by crop residue sterilization. Various studies have examined the influence of the sterilization methods, i.e. γ-irradiation, chemical fumigation, autoclaving, air drying [19,20] and treatment with sodium hypochlorite (NaOCl)/ethanol [21]. These techniques do not proceed in the same way and offer potential selectivity of both the location and degree of alteration generated. For example, while γ-irradiation led to the death of all microorganisms [22], the activities of residual enzymes (i.e. β-glucosidase) were still apparent for at least 8 weeks in soil [23]. A very recent study on microbial communities in maize has provided evidence that treatment with a well-balanced combination of sodium hypochlorite and ethanol (NaOCl/EtOH) is effective in isolating phyllospheric endophytes [24].
Our main objectives were therefore to determine the role of the initial residue community before its return to the soil, i.e. the roles of microorganisms and enzymes from the epiphytic and endophytic compartments in the soil decomposition process and the associated enzyme kinetics. Two sterilization treatments, involving γ-irradiation or sodium hypochlorite (NaOCl)/ethanol, were applied to maize leaves and roots and compared with a non-sterilized control. The treatments were chosen according to the following criteria: (1) γ-irradiation kills microorganisms but extracellular enzymes remain on the plant residue surface; (2) sodium hypochlorite (NaOCl)/ethanol is a surface sterilization treatment which removes microorganisms and related enzymes from the plant residue surface; (3) the non-sterilized control treatment which allowed the activity of all colonizing microorganisms and the associated enzymes.
Our hypothesis was that control (non-sterilized) treatments would sustain higher respiration and enzyme activities than sterilized treatments. Enzyme activity kinetics were studied in a set of enzymes composed of L-leucine aminopeptidase, cellobiohydrolase (CBH–1), xylanase, cellulase and laccase that arguably covers the major enzyme families involved in lignocellulose degradation in soil. Determination of the kinetics of these enzymes were performed on treated and control maize leaves and roots, before and at weekly intervals during the 43 days of plant residue incubation in soil.
2 Materials and methods
2.1 Soil sampling and processing
A silt loam soil with 77.3% silt, 17.8% clay, 3.8% sand, 0.95% organic C, and a pH (H2O) of 7.6 was sampled at the INRA experimental station (Somme, Mons-en-Chaussée) located in North East France (49° 80’ N, 3° 60’ E). After sampling, the soil was air dried for two days to reduce the moisture content to 120 mg H2O g−1 dry soil then sieved (< 2 mm) and cleaned manually to ensure complete removal of carbonates and organic crop residues [13]. The soil was then stored at 4 °C, prior to the incubation experiment, in order to limit microbial growth.
2.2 Plant materials
Leaves and roots of maize (Zea mays L.) cultivar Mexxal were harvested at physiological maturity from a field experiment performed at the INRA Lusignan experimental station (France). Leaf samples were therefore senescent and were separated manually from the stem after air-forced oven drying for one week at 30 °C. While the root samples were washed with 50 g L−1 sodium metaphosphate solution for 24 h, rinsed with deionized water to remove soil particles, then dried in an air-forced oven for one week at 30 °C.
Sodium hypochlorite/ethanol sterilization of the maize residues was performed as described by Reissinger et al. [25]. Maize leaves and roots were dipped separately in 1 L of a mixture of 100% sodium hypochlorite and 95% ethanol (1/1, v/v) for 1 min. The plant residue was then washed by dipping in deionized water to remove all chlorine, an active molecule derived from sodium hypochlorite (5 min max). A colorimetric test of chlorine concentration (Merck, Spectroquant) was performed on the water remaining after the final washing to check total chlorine removal. The leaves and roots were then air dried at 35 °C for 48 h.
For the γ-irradiation treatments, plastic bags containing chopped non-sterilized maize leaves and roots were sealed hermetically and received a dose of 45 KGy of γ-irradiation from a 60Co source (Ionisos, Dagneux, France). After sterilization, all plant residue samples were stored in sealed plastic bags in the dark at 4 °C until required for the incubation experiment and chemical analysis.
The maize leaves and roots for all treatments, i.e. non-sterilized leaves and roots (NSL & NSR), sodium hypochlorite-sterilized leaves and roots (HSL & HSR) and γ-irradiated leaves and roots (γSL & γSR), were chopped to approximately 0.5 cm length × 0.5 cm width and 1 cm length/3–5 mm diameter, respectively.
2.3 Chemical analysis
The chemical characteristics of maize leaves and roots were determined on two replicates of each sample. Total C and N contents of maize residues ground to 80 μm (Retsch, Cross Beater Mill), were measured by elemental analysis (NA 2000, Fisons Instruments, Milan, Italy). The cell wall contents of residues were determined as the neutral detergent fiber (NDF) according to the method described by Goering and Van Soest [26]. Briefly, the soluble fraction was removed by boiling 1 g of leaves or roots in deionized water at 100 °C for 30 min while the cytoplasmic components were removed by extraction with a neutral detergent solution at 100 °C for 60 min to obtain the NDF fraction corresponding to the cell walls. All residues from cell wall preparations were dried for 1 week at 30 °C and ground to 80 μm (Retsch, Cross Beater Mill).
Acid-insoluble residue (including lignin) and sugar contents were determined on plant residues before incubation. Lignin content was approximated as the acid-unhydrolyzable residue remaining after sulphuric acid hydrolysis of the cell wall polysaccharides, according to the Klason lignin (KL) determination [27]. Briefly, 50 mg of the relevant lyophilized residues were suspended in 0.5 mL of 12 M H2SO4 for 2 h at room temperature. Suspensions were then diluted to 1 M with deionized water, heated at 100 °C for 3 h and filtered. The remaining residues were dried at 105 °C and ash measurements made after 4 h at 500 °C. Klason lignin contents were calculated from the mass differences of samples before and after ash measurement.
The total sugar content of cell walls obtained from maize residues was determined before decomposition according to the method described by Blakeney et al. [28]. Ten milligrams of NDF samples were swollen in 125 μL 12 M H2SO4 for 2 h at 20 °C followed by acid hydrolysis with 1 M H2SO4 for 2 h at 100 °C. Monosaccharides released by the acid were separated by high performance anion-exchange chromatography (HPAEC) on a CarboPac PA-1 column (4 × 250 mm, Dionex, Sunnyvale, CA, USA), as described by Beaugrand et al. [29]. The monosaccharide composition was analyzed and quantified using 2-deoxy-D-ribose as internal standard and standard solutions of neutral carbohydrates (L-arabinose, D-glucose, D-xylose, D-galactose, L-rhamnose, D-mannose, L-fucose and D-fructose).
2.4 Experimental design
Non-sterilized and sterilized maize leaves (NSL, HSL, γSL) and roots (NSR, HSR, γSR), to be degraded separately in soil, were subjected to an incubation experiment using the methods reported in Bertrand et al. [7]. Potassium nitrate (61 mg N kg−1) was added to the initial soil concentration, equivalent to 9 mg N-NO3 kg−1 (no ammonium was detected), to ensure that N would not limit decomposition [30,31]. The concentration of N solution to be added was calculated by keeping the soil moisture at a potential of –80 KPa, corresponding to 190 g kg−1 air dry water content. Plant residues were mixed with soil samples at a rate of 2,000 mg C kg−1 dry soil and four replicates of each treatment were incubated at 15 ± 0.5 °C for 43 days. Soils with no added residue were prepared and incubated at the same time to serve as a control treatment. Soil moisture was maintained by weighing weekly throughout the incubation period and readjusting with deionized water when necessary. Carbon mineralization (C-CO2) was measured at regular intervals after the beginning of incubation, (after 3, 7, 14, 21, 28, 35 and 43 days) by placing 10 mL of 1 M NaOH in 250 mL glass jars to trap the CO2 released by soil samples (equivalent to 50 g dry soil). The CO2 concentrations trapped in the NaOH solutions were measured by continuous auto-analyzer (TRAACS 2000, Bran and Luebbe).
2.5 Enzyme extraction
After 0, 8, 14, 21, 28, 35 and 43 days of incubation, the maize residues and soil particles were manually separated to prepare leaf and root extracts. Day 0 corresponds to leaf and root residues that were thoroughly mixed with their relevant soils but removed after 10 min to prepare leaf and root extracts. Control extracts from non-sterilized residue, sodium hypochlorite- or γ-radiation-treated residues were obtained from residues that had not been added to soil. The maize leaves and roots were ground to 2 mm with a grinder (Retsch, Cross Beater Mill) and sodium acetate was used to extract proteins from the plant residue [32]. For each treatment, leaf and root extracts were prepared in 50 mM sodium acetate buffer, pH 6 to give a plant residue-to-buffer ratio of 1:125 [33]. After over-end shaking on a rotating shaker for 1 h, the suspensions were allowed to settle for 1 h before filtration through 0.45 μm filters (PTFE). The resulting plant residue extracts were used for enzyme activity measurements.
2.6 Enzyme assays
The activities of five enzymes were determined using fluorometric enzyme assays for L-leucine aminopeptidase (LAP) and cellobiohydrolase-1 (CBH–1) [33] or colorimetric enzyme assays for cellulase, xylanase [34] and laccase [35,36], according to previously reported methods. Enzyme assays were performed on the relevant leaf and root extracts to determine enzyme activities as equivalent absolute activity in enzyme units per gram dry matter (U g−1 DM). Enzyme activities were expressed in International Unit (U) which is defined as “the amount of enzyme activity which catalyzes the degradation of one micromole of the substrate per minute under standard conditions”.
2.6.1 Fluorimetric assay
The two substrates: 4-methylumbelliferyl-β-D-cellobioside (4-MUB-cellobiose) for CBH–1 activity measurement and 7-amino-4-methyl coumarin hydrochloride (7-AMC-leucine) for LAP activity measurement, as well as their respective fluorogenic compounds (4-MUB and 7-AMC) were obtained in crystalline form from Sigma-Aldrich. The fluorimetric assays were conducted in black 96-well microplates (NuncTm, FluoroNuncTm). A 200 μM stock solution was prepared either for the substrate (4-MUB or 7-AMC fluorogenic-conjugates) or for the fluorogenic group alone (4-MUB or 7-AMC) as catalysis products. Equivalent 200 μM of substrates were initially pre-dissolved in 1 mL of methanol, then made up to 50 mL with deionized water and kept in brown bottles at 4 °C. All further dilutions were made in a 50 mM sodium acetate buffer (pH 6). For each treatment of leaf or root extracts, 20 μL aliquots were pipetted into well microplates followed by 25 μL of 200 μM substrate solution and 80 μL of buffer to maintain the final volume at 125 μL well−1.
The assay plates with 6 analytical replicates by treatment were incubated in the dark (20 °C) for up to 3 h. The incubation times were based on preliminary assays of the linearity of the reaction over time. Reactions were terminated by adding 10 μL of 1 M NaOH to each well to raise the pH to 9, which corresponds to the optimal pH for fluorescence of these fluorogenics [37]. After excitation at 365 nm, the fluorescence emission intensity was measured at 460 nm by a computerised microplate fluorimeter (Spectra Max Gemini, Molecular Devices) which was programmed to shake the microplate for 5 s at 20 °C before reading, in order to homogenize the reaction medium. Enzyme activities of all the samples were determined in reference to a specific standard curve based on the appearance of fluorogenic products from the fluorogenic conjugates.
2.6.2 Colorimetric assay
Xylanase standards were prepared using the method reported in Biely et al. [34]. One gram of RBB xylan (Remazol Brilliant Blue R D-xylan, Fluka), which is a soluble chromogenic substrate for the assay of endo-1,4-β-xylanases, was dissolved in 80 mL of hot water (90 °C). The solution was then cooled at room temperature and the volume was adjusted to 100 mL.
For the cellulase assay, 2 g of powdered substrate (AZO-CM-Cellulose, Megazyme) were added to 80 mL of boiling water on a hot plate and stirred vigorously using a magnetic stirrer until apparent homogeneity (approx. 20 min). After cooling, 5 mL of 2 M sodium acetate buffer (pH 4.5) were added to the solution then the pH and final volume were adjusted to 4.5 and 100 mL, respectively. A solution was prepared to precipitate high molecular fragments of substrate (AZO-CM-cellulose) by dissolving 40 g of sodium acetate trihydrate and 4 g of zinc acetate in 200 mL of deionized water and adjusting the pH to 5 with 5 M HCl. The final volume of this precipitation solution was made up to 1 L with ethanol (95%) and stored at room temperature.
Laccase activity was determined as in the assays described by Buswell et al. [35] and Floch et al. [36]. One hundred milliliters of 0.55 mM of laccase substrate (ABTS, 2,2′-azinobis-3-ethylbenzothiazoline-6-sulfononic acid diammonium salt, Sigma) were prepared by using sodium acetate buffer (50 mM, pH 4.5) followed by vigorous shaking on a magnetic stirrer.
For each treatment, enzyme activities were measured in four replicates by adding 0.5 mL of residue extracts to 0.5 mL of the relevant substrate solution (RBB xylan/AZO-CM-cellulose) in the case of xylanase and cellulase, and 1 mL of residue extract to 0.5 mL of ABTS substrate solution in the case of laccase. All samples were incubated for 3 h at room temperature (20 °C). The added high molecular fragments of substrates (RBB xylan/AZO-CM-cellulose) were precipitated by adding 2 mL of ethanol (96% v/v) with vigorous shaking by vortex shaker (Heidolph, REAX 2000) while the low molecular weight fragments produced by enzymes remained in solution. Samples were then allowed to equilibrate at room temperature for 10 min before shaking and were then centrifuged at 5000 revolutions per minute for 5 min. The absorbance of the supernatant solutions was measured at 590 nm for xylanase and cellulase and at 414 nm for laccase, using a spectrophotometer (Heλios γ, Thermospectronic).
Xylanase and cellulase activities of all the samples were determined by reference to a standard curve obtained with purified endoxylanase from Thermobacillus xylanilyticus and commercial cellulase (322 U mL−1, endo-β-glucanase purified from Aspergillus niger, Megazyme) at concentrations in sodium acetate buffer (50 mM, pH 6) ranging from 0.01–0.1 U mL−1 and 0.001–0.01 mU mL−1, respectively. Laccase activity was determined on the basis of ABTS degradation by a purified laccase from Trametes versicolor (21.8 U mg−1, Sigma) used at concentrations ranging from 0.001–0.1 mU mL−1 in sodium acetate buffer (50 mM, pH 4.5).
2.7 Scanning electron microscopy (SEM)
Microbial colonization of the plant residue surface was observed by scanning electron microscope (SEM Philips XL 30). Leaves (0.2 cm length × 0.2 cm width) and roots (5 mm length × 2 mm diameter) were mounted on specimen stubs, with three replicates per sample. The residue surface was coated with a 15 nm layer of gold using a sputter coater (Balzers SCD 40) to make the residue surface electrically conductive and to prevent accumulation of a static electric charge on the specimens. The specimens were then transferred to the scanning electron microscope and observed using an acceleration voltage of 5 keV.
2.8 Data treatment and analysis
Carbon mineralization was calculated as the difference in CO2 released after each treatment between the residue-amended and control soils, and was expressed as a percentage of the added residue C.
Chemical features related to non-decomposed leaves and roots were expressed in relation to the initial dry matter (non-decomposed–dry matter, ND–DM) by taking into account the loss of mass residue by decomposition. This mass loss was calculated from the amount of C mineralized.
A two-way repeated measures ANOVA (rmANOVA) was used to determine the effects of residue type (leaves and roots) × treatments (γ-irradiated, surface-sterilized and non-sterilized) across time (until 43 days) on C mineralization kinetics and enzyme activities of L-leucine aminopeptidase, CBH–1, xylanase, cellulase and laccase. The rmANOVA examines the variation between type of residue and treatments as well as the variation within time, where each factor is considered in combination with time to produce factorial interaction terms. Since the effect of type of residue was always significant (P < 0.05), this was dropped from the analyses to examine more closely the effect of treatments over time. Thus for each type of residue, one-way repeated measure ANOVA (rmANOVA) were performed on dependent variables namely carbon and enzyme kinetics (LAP, xylanase, cellulase, CBH–1 and laccase) to interpret the effects of treatments interacting with time. The data were log or square root transformed to meet the assumptions of normality and homoscedasticity. Data analyses were performed with software Statistica 6.1 (Statsoft, Inc., 1984–2003).
3 Results
3.1 Initial chemical composition of non-sterilized and sterilized maize leaf and root
Initial total C, N and cell wall contents of maize leaf and root together with their sterilized treatments are indicated in Table 1. The total C contents of maize leaves did not vary much and ranged from 45.1 to 45.7% C. Indeed the significant changes measured were mainly due to a low variance (LSD value = 0.40). The C content in hypochlorite-sterilized root (HSR) was higher than in NSR and γSR. The total N content of maize leaf was 1.7 to 2.7 times higher than in root and sterilization treatments decreased the N contents of both leaves and roots compared to the corresponding non-sterilized samples. These variations in N content led to different C to N ratios: NSL gave the lowest C to N ratio which was three-fold lower than the highest value for HSR. Due to their lower N contents, the C to N ratios of the sterilized samples were higher than those of non-sterilized samples, especially in roots.
Total C, N and biochemical characteristics of non-sterilized and sterilized maize leaves (NSL, HSL, γSL) and roots (NSR, HSR, γSR) before soil decomposition (ND).
Variables | Total-C | Total-N | C to N ratio | Cell wall fraction | Total sugars | Glucan | Xylan | Klason lignin |
NSL | 45.1a | 1.88d | 24a | 73.4a | 66.7b | 36.7a | 24.1b | 12.5ab |
HSL | 45.7b | 1.59c | 29bc | 83.2b | 63.3ab | 34.3a | 23.2b | 14.4b |
γSL | 45.1a | 1.62cd | 28b | 73.6ab | 68.2b | 37.3a | 24.9b | 12.0a |
NSR | 46.5c | 1.07b | 43c | 84.1c | 57.6a | 34.5a | 16.8a | 16.3c |
HSR | 48.4d | 0.68a | 71e | 87.9d | 59.0ab | 35.7a | 18.8ab | 18.2d |
γSR | 45.9bc | 0.71ab | 65d | 83.6bc | 58.2ab | 34.9a | 19.3ab | 15.5bc |
LSD | 0.40 | 0.04 | 1.96 | 0.67 | 6.26 | 3.70 | 2.53 | 1.13 |
The cell wall contents were higher in non-sterilized roots than in leaves. Cell wall contents did not vary after γ-sterilization, whereas hypochlorite treatment resulted in significant enrichment in both leaf and root. Total sugar contents were slightly higher in leaves than roots, and were not significantly modified by the sterilization treatments. Glucose and xylose were the main monomers recovered after acid hydrolysis of samples, reflecting the presence of cell wall glucan and xylan, respectively. Both root and leaf contained approximately 35% glucan, and sterilization did not affect this level. The same was true for xylan content, but leaf xylan (close to 24% DM) was significantly higher than root xylan (mean of 18% DM). In contrast, Klason lignin contents were higher in roots than in leaves. Klason lignin was only significantly increased after hypochlorite treatment, in both leaf and root (Table 1).
3.2 Modification of plant residue surface induced by sterilization treatments, as observed by SEM
The presence of microorganisms and the effect of sterilization treatments on the outer surface morphology of maize leaves, roots and the respective sterilized treatments, before any contact with soil (controls), were observed by scanning electron microscopy (SEM) (Fig. 1a–f). Compared to roots (Fig. 1b), microbial colonization was more apparent on leaf surfaces where long and contrasting fungi hyphae or actinobacteria were observed (Fig. 1a). In contrast, smaller particles and possibly bacteria were more frequently found as speckles on the root surface (Fig. 1b). The epiphytic communities encountered on both leaf and root surfaces were drastically reduced after hypochlorite treatment (Fig. 1c, d). In addition to removing the microorganisms, this treatment concomitantly washed off almost all the surface particles on leaf (Fig. 1c), whilst some were still visible on root (Fig. 1d). Distinct patterns of apparent microbial colonization were observed after hypochlorite and γ-sterilized treatments. The leaf and root surfaces subjected to γ-radiation bombardment displayed probably dead and speckled particles (possibly bacteria), respectively (Fig. 1e, f) and were similar to the corresponding non-sterilized control samples in terms of epiphytic communities. Therefore, the surface appearance of the γ-irradiated samples was rather different from the clean surfaces observed after hypochlorite treatment. In general, no visible epidermal damage of the plant tissues or alteration of physical structure was apparent in the pictures of sterilized samples as compared to non-sterilized samples. For example, the leaf surfaces showed open stomata cells (Fig. 1a, c, e) whatever the treatment.
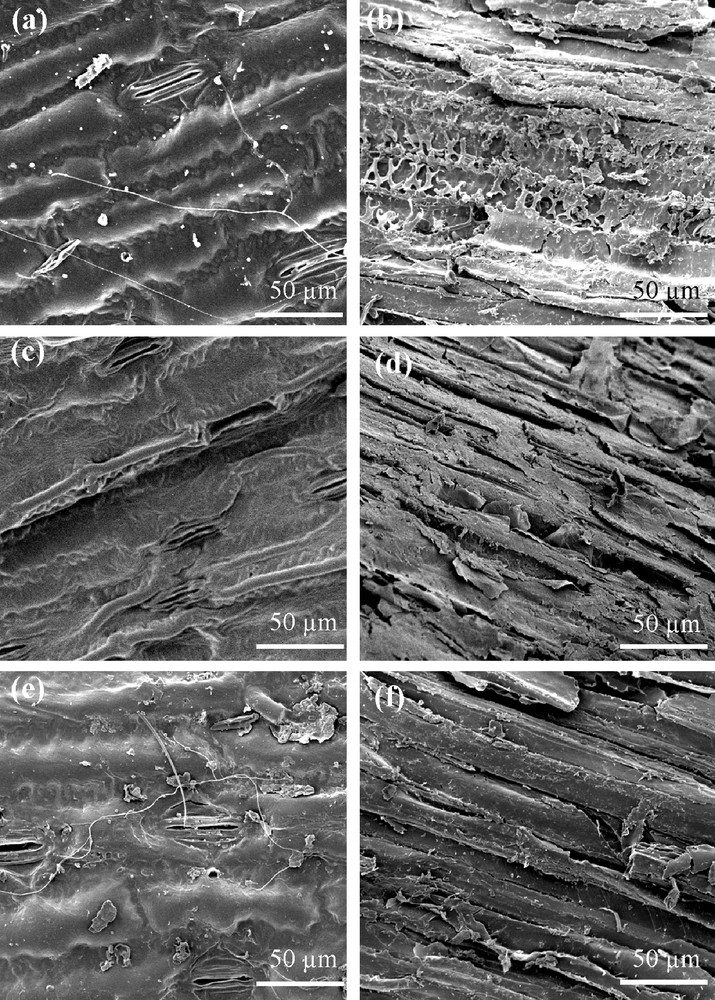
Scanning electron micrographs of representative surface observations from non-sterilized and sterilized maize leaves and roots. (a) and (b) represent non-sterilized maize leaf and root (NSL & NSR); (c) and (d) represent hypochlorite-sterilized maize leaf and root (HSL & HSR) and (e) and (f) represent γ-sterilized maize leaf and root (γSL & γSR), respectively.
3.3 C mineralization of maize leaf and roots
The amount of mineralized carbon in maize leaf- and root-amended soils varied markedly as a function of time (Fig. 2a, Table 3). In general, the main factor explaining the variance in carbon mineralized (% of carbon mineralized) was residue type (F = 90.01, P < 0.001) (data not shown) in which maize leaves (sterilized treatments included) decomposed more rapidly than roots (Fig. 2a). Depending upon the type of sterilization treatment, the amount of carbon mineralized did not vary significantly in case of maize leaves but was significantly different over incubation time as indicated by the ‘between’ P-values from repeated measures ANOVA (F = 28.97, P < 0.001) (Table 3). The amount of carbon mineralized was highest for non-sterilized treatments and lowest for gamma-sterilized treatments (Fig. 2a). Repeated measures ANOVA also indicated that the interaction of incubation time and sterilization treatments (T × Tr) had significant effects on the amount of mineralized carbon from both maize leaf- and root-amended soils.
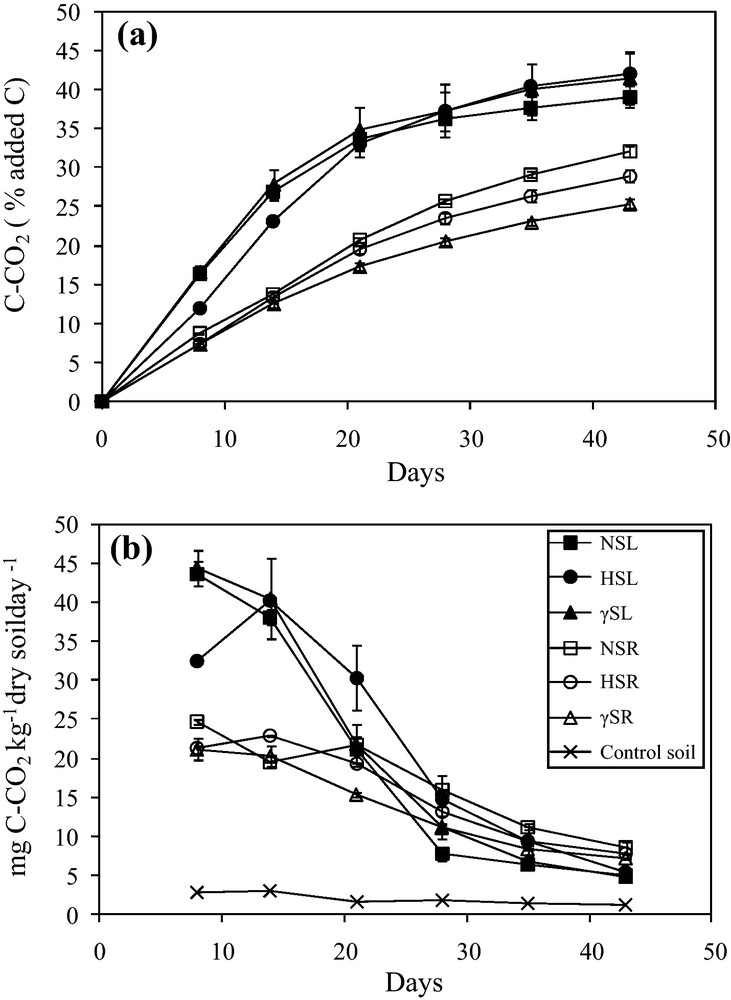
Cumulative carbon mineralization kinetics over time expressed as % added C (a) and carbon mineralization rates (b) measured in soil without maize residue (control) and after addition of non-sterilized, hypochlorite-sterilized and γ-sterilized maize leaves (NSL, HSL & γSL) and roots (NSR, HSR & γSR), respectively. Data are means of 4 incubation replicates (n = 4).
Statistical summary of repeated measures analysis of variance (rmANOVA) representing effects of sterilization treatments on C mineralization and enzyme kinetics.
% C min. | LAP | Xylanase | Cellulase | CBH–1 | Laccase | |||||||||
Residue type | Source of variation | df | F | P | F | P | F | P | F | P | F | P | F | P |
Maize leaf | Between-subject | |||||||||||||
Treatment (Tr) | 2 | 0.50 | 0.63 | 16.34 | 0.004 | 75.44 | < 0.0001 | 11.38 | 0.009 | 291.88 | < 0.0001 | 0.37 | 0.706 | |
Within-subject | ||||||||||||||
Time (T) | 6 | 827.73 | < 0.0001 | 7.17 | < 0.0001 | 181.05 | < 0.0001 | 246.35 | < 0.0001 | 757.50 | < 0.0001 | 118.74 | < 0.0001 | |
Interaction (T × Tr) | 12 | 6.12 | < 0.0001 | 2.78 | 0.009 | 9.29 | < 0.0001 | 4.16 | < 0.0001 | 22.93 | < 0.0001 | 1.93 | 0.064 | |
Maize root | Between-subject | |||||||||||||
Treatment (Tr) | 2 | 28.97 | < 0.001 | 16.26 | 0.004 | 27.14 | 0.001 | 12.64 | 0.007 | 284.24 | < 0.0001 | 23.33 | < 0.001 | |
Within-subject | ||||||||||||||
Time (T) | 6 | 7470.15 | < 0.0001 | 4.95 | < 0.001 | 404.93 | < 0.0001 | 188.34 | < 0.0001 | 79.73 | < 0.0001 | 15.04 | < 0.0001 | |
Interaction (T × Tr) | 12 | 15.72 | < 0.0001 | 3.27 | 0.003 | 1.37 | 0.226 | 1.20 | 0.320 | 6.78 | < 0.0001 | 4.72 | < 0.0001 |
Non-sterilized and γ-sterilized leaves (NSL and γSL) showed the same pattern of decomposition, in contrast to hypochlorite-sterilized leaves (HSL). However, almost the same amount of leaf carbon was mineralized for all three treatments up to the end of incubation with 39.1% ± 1.4, 41.5% ± 3.3 and 42.1% ± 2.8 of added C, respectively (Fig. 2a). In the case of HSL, an immediate effect of sterilization was observed which corresponded to a smaller amount of carbon mineralized from 0–14 days of incubation (23.1% ± 0.3 of added C), as compared to NSL and γSL (26.8% ± 0.9 and 27.8% ± 1.9 of added C, respectively). After this lag phase and until the end of incubation, HSL samples were mineralized in the same way as NSL and γSL (Fig. 2a). Roots gave rise to less cumulated C mineralized than leaves. No effect of sterilization was observed over the 0–14-day interval for roots, but the total amount of carbon mineralized (% of added carbon) up to the end of incubation, differed significantly and in the following order: NSR (32.1% ± 0.6) < HSR (28.9% ± 0.8) < γSR (25.4% ± 0.5) (Fig. 2a).
The rates of CO2 production in control soil, leaf (NSL, HSL, γSL) and root (NSR, HSR, γSR) are shown in Fig. 2b. In the control soil, the initial low rate of CO2 production decreased rapidly at the beginning of incubation and then became constant over a function of time (Fig. 2b). In the amended soils, the rates of CO2 release from leaf treatments were initially (8–14 days) much higher and faster than from roots but lower at the later stages of incubation, the cross-over point occurring during the 20–30-day period. Maximum rates for both leaf and root were attained at day 8, except for the hypochlorite-sterilized samples which showed a delayed maximum at day 14 of incubation. In the case of leaves, a rapid and maximum decrease of mineralization rate was observed during the 14–28-day period, while in the case of root treatments, this rate decreased continuously until the end of the incubation experiment (Fig. 2b).
3.4 Effect of sterilization treatments and time on enzyme kinetics
The effect of sterilization was examined by determining the initial enzyme activities on maize leaves, roots and the corresponding sterilized residues, before incubation in soil (Table 2). In general, significantly higher enzyme activities were found on leaf samples than on root samples except for L-leucine aminopeptidase (LAP). LAP activities did not change after γ-sterilization of either residue whereas LAP activity decreased significantly by 44% after hypochlorite treatment of roots (Table 2). γ-sterilization had no significant effect on xylanase, cellulase and laccase activities whereas hypochlorite sterilization led to a significant and strong decrease of these activities (Table 2). CBH–1 activities measured on leaves were significantly reduced after sterilization, especially after hypochlorite treatment, but no effect of sterilization treatments was observed on roots (Table 2).
Enzyme activities of L-leucine aminopeptidase, xylanase, cellulase, CBH–1 and laccase of non-sterilized and sterilized maize leaves (NSL, HSL, γSL) and roots (NSR, HSR, γSR) before adding to soil.
Variables | LAP | Xylanase | Cellulase | CBH–1 | Laccase |
NSL | 0.15b | 60.8d | 54.9c | 15.7c | 0.0072d |
HSL | 0.11ab | 4.9c | 6.9 ab | 0.7ab | 0.0059c |
γSL | 0.16b | 60.3d | 52.9c | 13.2b | 0.0070d |
NSR | 0.18b | 2.5b | 13.4b | 0.5ab | 0.0028b |
HSR | 0.10a | 0.2a | 2.4a | 0.1a | 0.0019a |
γSR | 0.15b | 2.1ab | 9.6ab | 0.1ab | 0.0023ab |
LSD | 0.03 | 2.01 | 8.02 | 0.66 | 0.0006 |
In general, all enzyme activities differed significantly ‘between’ the sterilization treatments for both maize residues with an exception of laccase in case of maize leaves (Table 3). At the same time, all enzyme activities were found to be significantly different over incubation time as indicated by the ‘within’ P-values from repeated measures ANOVA (Table 3). Repeated measures ANOVA also indicated that the interaction of incubation time and sterilization treatments (T × Tr) had significant effects on the enzyme activities except laccase in maize leaves and xylanase and cellulase in case of maize roots (Table 3).
On the basis of these differences, enzyme activities measured on non-sterilized and sterilized leaf and root extracts were plotted against the percentage of mineralized carbon to examine separately the effect of the initial residue microbial status on enzyme kinetics in context with sterilization treatments and incubation time (Fig. 3a–j).
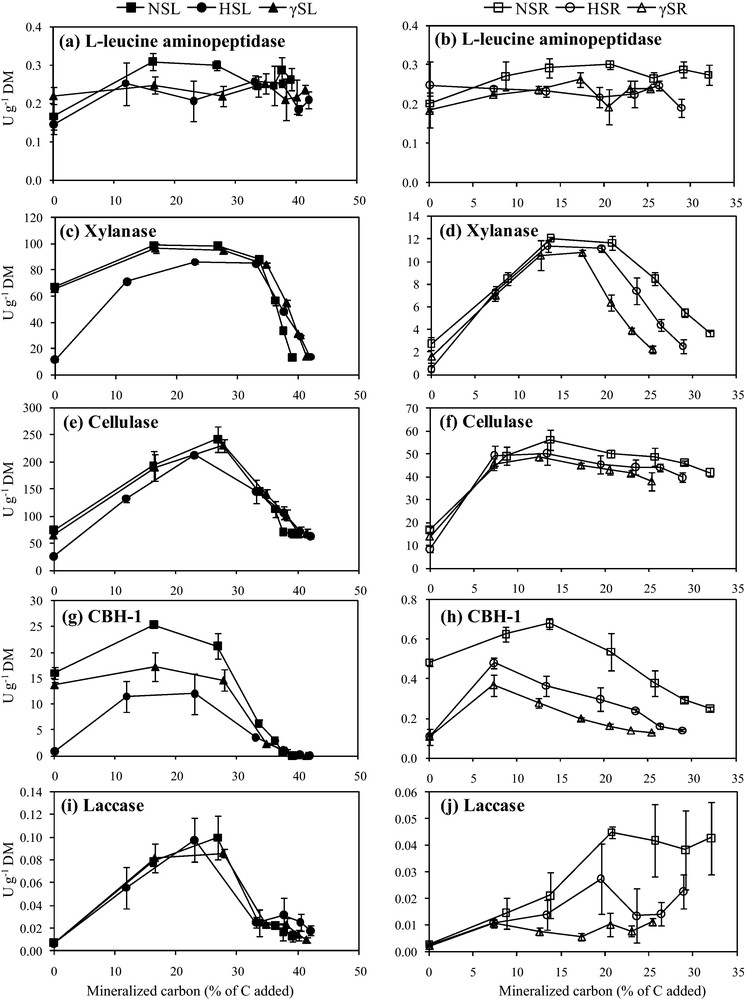
L-leucine aminopeptidase (a, b), xylanase (c, d), cellulase (e, f), CBH–1 (g, h) and laccase (i, j) activities of non-sterilized, hypochlorite-sterilized and γ-sterilized maize leaves (NSL, HSL & γSL) and roots (NSR, HSR & γSR) treatments represented as a function of mineralized carbon (% of C added), respectively. Enzyme activities were expressed as enzyme units per gram dry matter (U g−1 DM). Error bars represent the standard error of the mean of four replicates (n = 4).
L-leucine aminopeptidase activities, which mirror a major protease family, differed significantly by sterilization treatments for maize leaves (F = 16.34, P < 0.01) and roots (F = 16.26, P < 0.01) (Table 3) and were relatively low in both leaf and root treatments (between 0.1–0.4 U g−1 DM) at time 0 (Fig. 3a, b). When the respective residues were added to soil, maximum L-leucine aminopeptidase activities were obtained for NSL and NSR when 38% and 29% of C were mineralized, respectively. The measurements of L-leucine aminopeptidase enzyme activities on all the sterilized samples indicated that the HSL, γSL, HSR and γSR values were lower than NSL and NSR and remained similar till the end of incubation (Fig. 3a, b).
Xylanase activities which provide an indication of enzymatic degradation of the major cell wall heteroxylan, varied significantly for sterilization treatments in case of maize leaves (F = 75.44, P < 0.0001) and roots (F = 27.14, P < 0.001) being greater on leaf treatments than root treatments (Table 3; Fig. 3c, d). Xylanase activities were similar in NSL and γSL from the start to end of incubation, attaining a maximum when 28% of C was mineralized (Fig. 3c). In contrast xylanase activities in HSL were 4 times lower at the start but finally joined the enzyme activity curve for NSL when 34% of C was mineralized (Fig. 3c). In case of roots, xylanase activities of NSR showed 2 to 5 times more xylanase activity than γSR and HSR. Maxima were reached when 13% of C was mineralized, i.e. earlier than in leaves. After this maximum, the root treatments ranked as NSR > HSR > γSR and followed the same trend until the end of incubation (Fig. 3d).
Cellulase activities of maize leaves and roots were also significantly affected by sterilization treatments (F = 11.38, P < 0.01; F = 12.64, P < 0.01, respectively), being greater in leaf treatments as compared to root treatments (Table 3; Fig. 3e, f). Cellulase activities of leaf extracts showed almost the same patterns as xylanase activities, with HSL extracts showing 2.5 less cellulase activity than NSL and γSL at time 0 (Fig. 3e). Cellulase activities in HSL remained lower until 33% of C was mineralized. Cellulase activities measured on hypochlorite-treated roots were approximately 2 times lower at time 0 than under other conditions, and reached their maximum earlier when only 8% of the C was mineralized. In contrast to leaves, the patterns of cellulase activity measured in NSR and γSR differed from those of xylanase activity and the decrease until the end of incubation was smaller (Fig. 3f).
CBH–1 activities of maize leaves and roots varied significantly across the sterilization treatments (F = 291.88, P < 0.0001; F = 284.24, P < 0.0001, respectively), being lower in root treatments as compared to leaf treatments (Table 3; Fig. 3g, h). CBH–1 activities were considerably decreased by sterilization treatments in both leaves and roots (Fig. 3g, h) and remained 10 times higher for leaves than for roots. At time 0, CBH–1 activities measured on NSL were 20 and 1.2 times higher than on HSL and γSL, the extent of variation being much greater than with cellulase (Fig. 3e). In leaves, CBH–1 activities reached their maximum more rapidly than cellulase and the difference between treatments remained significant until 35% C was mineralized. Almost no CBH–1 activity was observed in leaf extracts at the end of incubation (Fig. 3g). CBH–1 activities on roots did not follow the cellulase patterns (compare Fig. 3h and Fig. 3f) and were comparable to those on leaves. At time 0, NSR showed 4.5 times more CBH–1 activity than HSR and γSR (Fig. 3h). Maximum CBH–1 activities in NSR were obtained when 14% of C was mineralized, in contrast to the activities in both HSR and γSR for which maxima were reached when 7% of C was mineralized (Fig. 3h).
Sterilization treatments did not have significant effect on laccase activities of maize leaves but did significantly affect laccase activities of maize roots (F = 23.33, P < 0.001) (Table 3), resulting in greater laccase activities in leaf treatments than root ones (Fig. 3i, j). In contrast to the other enzyme activities tested, laccase activities in root and leaf extracts at time 0 were comparable, so no significant variations were observed between treatments. After the start of incubation when 12% of C was mineralized, a transitory period of less activity was determined for HSL, as compared to NSL and γSL, while no difference between treatments was observed during the remaining period of incubation (Fig. 3i). For roots, lower laccase activities were observed on sterilized samples when 6–8% of C was mineralized (Fig. 3j). After this stage, γSR activities remained parallel as a function of mineralized C while the NSR and HSR activities increased until the end of incubation (Fig. 3j).
4 Discussion
The effect of adding residues of contrasting quality (i.e. roots versus leaves) on C mineralization kinetics and enzyme dynamics was discussed in a previous paper [38]. In that study we demonstrated that the changes in activities of polysaccharide-degrading enzymes provided an exact reflection of decomposition and that most of the relevant biological activity was concentrated on the residue, in agreement with a recent report [39]. The validity of these conclusions is strengthened by the present study since higher levels of C mineralization and enzyme activities were monitored in leaves than in roots, independently of the sterilization treatments. In the present study, we focused on the impact of initial residue colonization in an attempt to separate the role of epiphytic activities, i.e. those on the external surface of the residues, from endophytic activities which occur within the residue. Our hypothesis was that the impact of residue-colonizing microorganisms could be assessed by subtracting the effect of γ-irradiation from non-sterilized treatments, while insight into the specific contribution of endophytes might be obtained by subtracting the effect of hypochlorite treatment (i.e. surface sterilization) from non-sterilized treatments.
4.1 Interactions between residue quality and sterilization treatments
Surface sterilization has frequently been applied to eliminate surface-colonizing microbial populations of fungi and bacteria from plant residue [40,41]. SEM comparisons of hypochlorite-sterilized treatments and non-sterilized treatments, confirmed that the microorganisms which were precursors of enzyme production were completely removed from leaf and root surfaces without any visible physical damage to the residue surface (Fig. 1c, d). On the contrary, γ-sterilized leaf and root treatments revealed the presence of dead microorganisms and debris (e.g. fungal hyphae or actinobacteria) on the residue surfaces which presented the same physical features as in the non-sterilized treatment. Total N contents in both residues were reduced after hypochlorite sterilization, resulting in a slight increase in cell wall, C and Klason Lignin contents. These hypochlorite effects can possibly be attributed to the removal of soluble compounds by a ‘washing effect’ during dipping of the plant residue in the NaOCl/ethanol solution. This also suggests that residue-colonizing microorganisms have assimilated a portion of N which is removed with their elimination from the residue surface. Accordingly, it has been shown [13] that in maize roots, microorganisms (bacteria and fungi) are rich in N, as compared to the cell wall. Nevertheless some N could also correspond to the soluble proteins removed by the washing effect of hypochlorite treatment. Sodium hypochlorite/ethanol sterilization was considered to remove surface-colonizing microorganisms, however, given the significant decrease of enzyme activities in hypochlorite-treated leaves and roots (Table 2), we hypothesize that such a treatment would concomitantly wash off enzymes and some soluble compounds in addition to removing the surface-colonizing microorganisms (Fig. 1c, d; Table 1).
In contrast, γ-irradiation had no significant effect on the chemical composition of either leaf or root residues, as γ-irradiated residues were not immersed in any liquid during the sterilization process. To ensure complete inactivation of the microorganisms, a 45 kGy dose of γ-irradiation was used, as 25 kGy is sufficient to eliminate all fungi and bacteria (except radioresistant bacteria) [42] and is also currently used in industrial food and medical sterilization processes. The selected γ-irradiation dose is considered to kill all microorganisms while conserving extracellular enzyme activities and protein functions [23]. Accordingly, the treatment used did not significantly affect the enzyme activities measured in leaf and root extracts (Table 2). Nevertheless some proteins may be denatured more rapidly, depending on local water conditions [43,44], so no generalization can be made. Structural proteins are reported to be less solvated in γ-irradiated samples which may impact their function [45].
4.2 Impact of colonizing microorganisms on enzyme activities and residue decomposition
Non-sterilized and γ-treated leaves reached the same level of cumulated C mineralization after 43 days of incubation, suggesting that residue-colonizing microorganisms, whether endophytic or epiphytic had no effect. In contrast, hypochlorite-treated leaves mineralized at a slower rate during the first 7 days of incubation (Fig. 2), which can be explained by the decrease in HSL soluble components (10% DM) compared to NSL and γSL, as previously demonstrated [5,6,38,46]. The enzyme activity patterns (xylanase, cellulase, CBH–1), following incubation of residues in soil, indicated that leaf samples were mostly affected by hypochlorite treatment, in contrast to roots, suggesting a greater presence of those enzymes on the leaf surface and/or in the soluble fraction. Hypochlorite treatment was followed by washing until no chorine was detectable, so the weaker activities of xylanase, cellulase and CBH measured on leaves during their mineralization should not be due to a residual toxic component. In this respect, laccase activities displayed similar patterns during the decomposition of non-sterilized and sterilized leaves.
The cumulated amount of C at day 43 for roots ranked as follows NSR > HSR > γSR. Hypochlorite treatment reduced the amount of soluble components to a much weaker extent in roots than in leaves, possibly due to the lower liquid permeability of roots. Therefore the observed decrease in C mineralization between non-sterilized and hypochlorite-treated roots (about 10% of added C) would be mainly due to colonizing microorganisms. However, no differences in the enzyme kinetics of sterilized roots were recorded until 15% C was mineralized except for CBH–1 (Fig. 3). CBH–1 is an accessory exo-cellulase which assists microbial assimilation by catalyzing the 2–4 terminal units at one end of the polysaccharide chain releasing cellobiose. These enzymes work progressively either on the reducing end or the other end. Further, β-glucosidase hydrolyzes the cellobiose units in glucose monomers. The enzyme dynamics during the first step of decomposition suggest that oligoglucosides were present in the soluble fraction of roots on which the epiphytic and endogenic microorganisms were acting. Few studies have described the spectrum and activity of endophytes colonizing roots at the senescent stage. It has already been found [47] that endophytic microorganisms in sugar beet promote growth and increase beet yield but no published data exist for plants after harvesting. The variation in C mineralization and enzyme dynamics between non-sterilized and sterilized roots suggests that the activities of epiphytic and endogen microorganisms would be of the same order of magnitude, assuming that γ-irradiation killed all microorganisms [22] but left enzymes active. It is possible that the entire residue structure would be slightly altered by the treatments. Cellulose and hemicellulose have been shown to be less recalcitrant to enzymatic hydrolysis after high dose γ-irradiation [48]. In our case after irradiation at much lower dose (45 KGy), lower mineralization rates were observed with roots, and no variation could be detected for leaves. Hence, the enzyme activities measured in γ-irradiation-treated roots were reduced throughout almost the entire incubation period (Fig. 3). This fact plus the major differences between our treatment and those in the above-cited reports, i.e. γ-irradiation dose and in vitro degradation of polymers, permit us to assume that the cell wall would be slightly modified without any strong impact on the overall mineralization dynamics and would thus permit treatment comparisons.
L-leucine aminopeptidase (LAP) activities in leaf and root samples were slightly affected by the sterilization treatments. These proteases did not vary much with residue decomposition in soil (Fig. 3) as a function of mineralized carbon, possibly due to the non-limiting soil nitrogen conditions.
5 Conclusions
Firstly, no effect attributable to any kind of colonizing microorganisms, epi- or endophytic, on C mineralization kinetics and enzyme dynamics was observed on leaves. However the side effect of hypochlorite highlighted the key role of soluble compounds during short-term decomposition in soil.
Secondly, the significant role of colonizing microorganisms and enzymes on C mineralization of roots was demonstrated. These underground tissues, as well as their original locations, are more exposed to microorganisms (rhizosphere). Overall, colonizing microorganisms whatever their location, will promote post-harvest decomposition. In addition, epiphytic and endophytic microorganisms of roots act in the same way during the soil decomposition process.
The major differences in C mineralization, observed in roots versus leaves, could be attributed in part to variations in the colonizing microorganism communities and enzyme pools initially present on the surface or within the residue structures, while specific chemical characteristics explain the main differences. Nevertheless the influence of residue morphology, i.e. tissue architecture and cell dimensions, in trapping enzymes and enabling microorganisms to colonize plant residues still requires further investigation.
Disclosure of interest
The authors declare that they have no conflicts of interest concerning this article.
Acknowledgements
This work was supported financially by the French National Institute of Agronomic Research (INRA), Reims, France and the Higher Education Commission (HEC) of Pakistan. We would like to thank Dr René Guenon for the help with statistical analysis. We would also like to thank Gonzague Alavoine, Sylvie Millon and Olivier Delfosse for their technical assistance.