1 Introduction
As a result of the release of anthropogenic CO2 in the atmosphere, temperatures worldwide have been increasing, and ocean pH has been decreasing [1]. These two stressors may have dramatic effects on coral reefs [2,3]. The seawater temperature in the tropics has already increased of almost 1 °C over the past hundred years, and the increase is still continuing [4]. High seawater temperatures have been reported as the principal factor inducing coral bleaching, the loss of the coral photosymbionts [5]. The increase of CO2 in the atmosphere results in an increased absorption of CO2 by the ocean. The associated chemistry, the hydrolysis of CO2, causes the pH of water to decrease. The pH of seawater is already 0.1 pH unit lower than pre-industrial values and may decrease another 0.3–0.4 unit by the end of the century if the present trend in CO2 emissions continues [1]. The change in pH and the increase in dissolved inorganic carbon shift the carbon balance, resulting in a lower aragonite saturation state (Ωaragonite). Calcifying organisms may be impacted by this change. Decreases in Ωaragonite have been shown to decrease the calcification rate of certain organisms [6–8]. It has been predicted that a doubling of the pre-industrial atmospheric CO2 concentration may result in a 10–50% decrease of the calcification rate of reef-building corals [9]. However, the effect of acidification on the calcification process is still unclear. Calcification in scleractinian corals occurs in an extracellular calcifying fluid located between the calicoblastic cell layer and the skeleton [10]. Corals maintain a high Ωaragonite at the calcification site, and this process requires energy [11–13]. A lower pH in the surrounding water could therefore increase the consumption of the energy used to maintain an elevated pH in the sub-calicoblast space [14].
Corals and other benthic communities change the carbonate chemistry of the seawater flowing through coral reef ecosystems [15]. Moreover, corals are complex organisms, within which microenvironments with different chemical characteristics can be described [16,17]. The microenvironment surrounding corals can differ greatly from the environment seen at a larger scale in the surrounding waters [18,19]. Diffusion limitations in the layer around an organism create a diffusive boundary layer (DBL) on the surface of the organism. The chemistry of the DBL is highly influenced by the organism's metabolism and shows diurnal variations according to changes in mainly photosynthetic and respiratory activity. The oxygen concentration varies from undersaturated during the night to hypersaturated during the day. The pH also shows strong variations between day and night, with values clearly differing from the surrounding water [20]. To our knowledge, changes in the chemistry of the DBL under different stresses have only been the subject of one study, and only high-temperatures were tested [21]. Up-regulation of the pH at the interface between tissue and skeleton leading to higher pHs compared to the surrounding seawater even under acidified conditions [12,22], highlights the need to study the effect of stressors on the microenvironment within corals to understand the effects that changes in the surrounding environment will have on corals. In this study, the effects of high-temperature stress, acidification and a combination of both factors on coral metabolism and on the chemistry of the DBL were investigated using microsensor techniques.
2 Materials and methods
2.1 Biological materials
Individual polyps were obtained from one parent colony of Galaxea fascicularis kept for several years in an outdoor aquarium with running seawater. The seawater is pumped from the reef in front of the Sesoko marine station, Tropical Biosphere Research Center, Okinawa, Japan. The average temperature, salinity, pH and alkalinity for the month preceding the experiment were: 24.8 °C, 33.2, 8.07, and 2.28 mmol kg−1, respectively. The maximum recorded temperature was 27.1 °C (record of coastal observation at Sesoko station, Tropical Biosphere Research Center, the University of the Ryukyus). The individual polyps were allowed to recover for 1 week, and 32 polyps were transferred to four separate indoor aquariums with running seawater and a 12:12 light cycle, with a light level of 200 μmol s−1 m−2. The polyps were fixed on a rubber stand to place their body wall at an angle of 45°.
2.2 Incubation conditions
The polyps were incubated under four different conditions: 27 °C/400 ppm CO2, 27 °C/750 ppm CO2, 32 °C/400 ppm CO2 and 32 °C/750 ppm CO2 (n = 8 for each treatment). The polyps at 27 °C, which was the maximum temperature at which corals were exposed during the month preceding the experiment, were incubated for five days at both pCO2 values, and the polyps at 32 °C were incubated for 1 day at both pCO2 values in combination with the high-temperature. Polyps at both pCO2 showed no signs of stress at 27 °C and were fully expanded throughout the incubation and measurement period (5 days). However, the polyps incubated at 32 °C showed signs of bleaching (discoloration) and stress (retraction of the tentacles) after one day. For this reason, the measurements at this temperature were performed after only 24 h of incubation. Each aquarium had a volume of 9 L and was supplied with unfiltered seawater at a flow rate of 150 mL min−1. pCO2 levels were established by injecting CO2-saturated seawater (100%) [23] with a peristaltic pump until the desired pH was obtained. The flow rates were adjusted to reach a pH corresponding to approximately 750 ppm CO2 in each high-CO2 treatment. CO2-saturated seawater was prepared by bubbling pure CO2 gas into natural seawater for 1 h. The pH was measured at the beginning of the incubation with an Orion 4 stars pH sensor equipped with an 8156BNUWP pH electrode (Thermo scientific, Inc., USA) calibrated with a standard National Bureau of Standards buffer solution (Mettler Toledo, Inc). The 400-ppm treatment corresponded to the ambient CO2 levels. The variation in pH of the incubation water was checked on a daily basis in the morning. The pH in the aquarium varied within 0.05 pH unit. Samples for total alkalinity in each aquarium were taken in the morning on day 5 for 27 °C and day 1 for the 32 °C treatment. Seawater was filtered with a syringe filter (0.45 μm) and kept cool until analysis. Total alkalinity was measured by potentiometric titration with HCl at 0.1 mol L−1 with a Metrohm titrator (785 DMP titrino), computation was done using the Gran plot method [24]. Reproducibility of the measurement was ± 2 μmol kg−1 (1σ, n = 10). A working seawater standard was used for the calibration of the measurement. This standard was analyzed precisely for total alkalinity with a certified reference material for oceanic CO2 measurement (CRM Batch #50) distributed by A. Dickson of the Marine Physical Laboratory, University of California, San Diego. The conditions obtained in each treatment are summarized in Table 1. The characteristics of the carbonate system in the seawater were calculated using the apparent equilibrium constants K’0 from Weiss (1974) [25] and K′1 and K′2 from Mehrbach and Culberson (1973) [26], as described by Millero (1979) [27] and Casareto et al. [28]. The aragonite saturation state (Ωaragonite) was calculated from the calcium (Ca2+) and carbonate (CO32−) concentrations as Ωaragonite = [Ca2+] [CO32−]/K′sp. K′sp is the stoichiometric solubility product of aragonite, derived from a function of salinity and temperature [29]. Calcium was fixed as a function of salinity (33.2) at a value of 9.75 mmol kg−1.
Incubation conditions characterizing the carbonate system in the different treatments.
pH | Alkalinity (mmol kg−1) | Temp (°C) | [CO32−] (mmol kg−1) | [HCO3−] (mmol kg−1) | [CO2(aq.)] (mmol kg−1) | TIC (mmol kg−1) | pCO2 (ppm) | Ωaragonite | |
Low temperature | |||||||||
Low pCO2 | 8.149 ± 0.036 | 2.21 | 27.4 ± 0.3 | 0.19 | 1.73 | 0.01 | 1.94 | 446 | 3.138 |
High pCO2 | 7.962 ± 0.035 | 2.21 | 27.2 ± 0.4 | 0.14 | 1.87 | 0.02 | 2.03 | 753 | 2.192 |
High-temperature | |||||||||
Low pCO2 | 8.146 ± 0.042 | 2.22 | 32.0 ± 0.5 | 0.21 | 1.71 | 0.01 | 1.93 | 474 | 3.518 |
High pCO2 | 7.972 ± 0.028 | 2.23 | 32.0 ± 0.5 | 0.15 | 1.85 | 0.02 | 2.02 | 786 | 2.555 |
2.3 Microsensor measurements
Microsensor measurements were performed in a custom flume with a laminar flow of 5 cm s−1 (Fig. 1). The total volume of the flume and reservoir was 3 L. Water was changed after each measurement. Water from the respective incubation aquariums was used to fill the flume. For the measurement at 32 °C, the flume was placed in a heated water bath (32 ± 0.5 °C). Oxygen, pH and temperature were monitored in the flowing water during the measurements, using an Orion 4 star pH/DO meter placed at the end of the flume. No substantial changes occurred in the oxygen and pH levels in the flowing water during metabolic rates’ measurements of each polyp. Light at 200 μmol s−1 m−2 was provided by a metal halide lamp with an angle of incidence of 45° to the wall of the polyp. Oxygen microsensor (oxygen micro-optode, type PSt1, Presens Gmbh, Regensburg, Germany), and a pH microsensor (pH micro-optode, Presens Gmbh, Regensburg, Germany) were mounted on a manually operated Märzhäuser micro-manipulator (MM33, Sutter Instruments, 10-μm resolution). Microsensors were calibrated as indicated by the manufacturer. Briefly, oxygen microsensors were calibrated using a 0% oxygen solution made by dissolving 1 g of sodium sulfite in 100 mL of distilled water and a 100% oxygen solution made by bubbling air in 100 mL of distilled water. pH microsensors were calibrated using the multipoint calibration method, the different pH used were 5.0, 6.0, 7.0, 8.0, and 9.0. The solutions at different pH were prepared just before calibration by adjusting the pH of seawater with HCl or NaOH. The measurements were performed on the body wall of the polyps at an angle of 90°.

Schema showing the set-up used for microsensor measurements. Individual coral polyps were fixed on rubber stands and placed in a custom flume under a 5-cm s−1 current. Light at 200 μmol s−1 m−2 was provided by a metal halide lamp.
The thickness of the DBL in the flume conditions was measured on five polyps, with three measurements per polyp. The polyps were maintained at 27 °C/400 ppm. The variations in oxygen concentration as a function of the distance from the polyps were used for estimation of the DBL thickness The net photosynthetic rates and dark respiration rates were calculated by measuring the flux of oxygen under an electrode placed at the surface of the corals (n = 3 polyps, with 10 measurements per polyp). Fick's law of diffusion was used to calculate the oxygen fluxes [30]. The parameters used were the oxygen concentrations at the surface of the polyp and outside the DBL, the average DBL thickness and the temperature-corrected diffusion coefficients. The oxygen diffusion coefficients used were –ΦDs = 2.3525 10−5 cm2 s−1 at 27 °C and –ΦDs = 2.6318 10−5 cm2 s−1 at 32 °C [31,32]. For each polyp, the oxygen at its surface was measured at ten different locations on the up-front body wall. The oxygen fluxes were calculated for each location. The measurements were performed under light and dark conditions after a minimum of 5 min of adaptation after the change in luminosity. The results were analyzed using a two-way ANOVA mixed model with the polyp factor used as a random factor. The pH in the DBL in light and dark conditions was measured for the polyps at 27 °C and at both pCO2 values by placing the microsensor tip at the surface of the polyps. Minimum and maximum values of pH were recorded once pH is stabilized. The significance of the difference of the pH values in the DBL under the two different pCO2 were evaluated using a pairwise t-test.
2.4 Tissue ATP concentration analysis
Tissue homogenates were prepared with the air-jet method, in which MilliQ NaCl (30 g L−1) with formaldehyde at a final concentration of 5% was sprayed using a paint sprayer ST (Asahi pen, Japan) to strip the tissue from the coral skeleton. The tissue slurry was then centrifuged at 3000 g for 10 min to separate host and zooxanthellae, and ATP was measured in the supernatant (host fraction) using an ATP auto-analyzer (TOA DKK, AF-100) after 1000 times dilution, as described by the manufacturer. The protein content was also determined in the supernatant by the Bradford method [33] after dilution. Results were analyzed using a two-way ANOVA.
3 Results
The DBL thickness was found to be 370 ± 90 μm (average ± SD, n = 15) and ranged from 250 to 500 μm (Fig. 2).

Dissolved O2 profiles used for the determination of the thickness of the DBL. Each symbol represents the measurement for a different polyp. The vertical dashed line represents the average thickness of the DBL in this experiment; the gray area is its standard deviation.
The net photosynthetic rates (Fig. 3, A) decreased significantly in the high-temperature stress treatment (DF = 8, P < 0.05) but did not change significantly in response to the increased partial pressure of CO2 (DF = 8, P = 0.31). No significant interaction was observed between temperature and pCO2 treatments (DF = 8, P = 0.096). The net photosynthetic rates were 0.25 ± 0.08 μmol O2 cm−2 h−1 and 0.18 ± 0.10 μmol O2 cm−2 h−1 (average ± standard deviation, n = 6, 10 measurements each) at 27 °C and 32 °C, respectively.

Net photosynthetic rates (A) and dark respiration rates (B) as measured by oxygen fluxes in the light and dark, respectively. Respiration rates were corrected to positive values. The error bars indicate standard deviations among all measurements, n = 3, 10 measurements each.
The respiration rates (Fig. 3, B) were low for all corals and close to zero for the polyps incubated at high-temperature. These low rates made the measurements difficult. The average ± standard deviation respiration rates (n = 6, 10 measurements each) for the different temperature treatments were: 0.08 ± 0.07 and 0.00 ± 0.04 for 27 °C and 32 °C, respectively. The respiration rates decreased significantly in response to temperature stress (DF = 8, P < 0.05). CO2 did not significantly affect respiration rates (DF = 8, P = 0.41) and no interactions were observed (DF = 8, P = 0.60).
The variations in pH in the DBL, measured with the electrode placed in contact with the polyp, were recorded over the light and dark phases for the polyps incubated at 27 °C (Fig. 4). The pH generally stabilized after 5 min, and the stabilized value was recorded. The maximum pH was recorded under light conditions, the minimum pH under dark conditions (Fig. 5). The minimum pH (average ± SD) at the surface of the polyp, obtained during dark conditions, was significantly lower at 750 ppm, 7.5 ± 0.1, compared with 400 ppm, 7.9 ± 0.1, (t-test, P = 0.01, n = 3). In contrast, the pH in the DBL did not differ significantly between the CO2 treatments in the light condition, with pH values of 8.7 ± 0.3 and 8.5 ± 0.3 for 400 and 750 ppm CO2, respectively (t-test, P = 0.66, n = 3).

Examples of pH dynamics at the surface of isolated polyps incubated at 27 °C under two different pCO2: (A) 400 ppm and (B) 750 ppm. Gray and white areas represent dark and light periods, respectively.
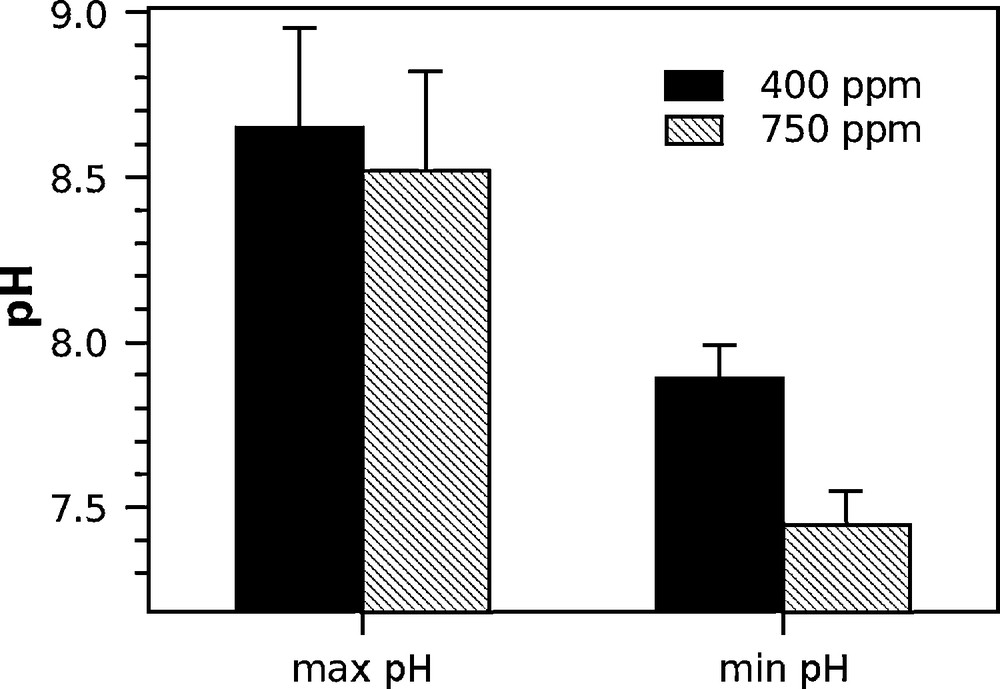
pH measured in the DBL of isolated polyps incubated at 27 °C under two different pCO2. Error bars indicate standard errors. Max pH indicates the average value for three polyps in each treatment of the maximum stabilized pH reached in the DBL under light conditions. Min pH represents the average value of the minimum stabilized pH reached in the DBL under dark conditions. Examples of the dynamics of the pH in the DBL under light/dark shifts are shown in Fig. 4.
High-temperature stress produced a significant decrease in the host ATP content (DF = 1, P < 0.05) (Fig. 6). High pCO2 had no significant effect at the 95% interval of confidence on host ATP contents (DF = 1, P = 0.06). The average (± standard deviation, n = 10) of host ATP contents were 3.67 ± 1.49 nmol mg prot−1 and 2.65 ± 1.08 nmol mg prot−1 for 27 °C and 32 °C respectively. No significant interaction between treatments could be observed (DF = 1, P = 0.18).

Host ATP content in isolated polyps (n = 5) for each treatment. Error bars indicate standard deviation among replicates.
4 Discussion
High-temperature stress had a strong negative effect on the metabolism of isolated polyps. Signs of bleaching were visible after 24 h and concurrently the net photosynthetic rates were reduced. High variability in oxygen measurements was observed, but the photosynthetic rates found in the control treatment of this study are in agreement with rates from a previous study [34]. This variability may be due to the difference in the DBL thickness. Thickness of the DBL may vary with the shape of the polyps and with the location on the polyp where the microsensor was placed. Different chlorophyll contents among the polyps in the same treatments may also influence the observed rates. No significant interaction on photosynthesis rates of temperature and pCO2 could be shown at the 95% confidence level; however, the decrease in photosynthesis seemed of less amplitude under combined high-temperature and high pCO2 compared to high pCO2 alone. This difference may indicate that higher partial pressure in CO2 may mitigate the negative effect of high-temperature on the photosynthetic rates. It has been reported that zooxanthellae use HCO3−as a source of inorganic carbon [35]. We manipulated the pCO2 of seawater in the incubation vessels by adding CO2-saturated seawater. This addition produced a slight increase in the concentration of bicarbonate ions. The enhanced photosynthetic rates at high-CO2 only occurred at high-temperatures. It is possible that the zooxanthellae were only limited by the amount of CO2 at this temperature and that other factors limit the photosynthetic rate of zooxanthellae at normal temperatures [36]. Zooxanthellae also use CO2 produced through respiration, as previously shown by Al-Horani et al. [11]. Under high-temperature stress, host respiration was decreased and therefore the supply of CO2 to the zooxanthellae also decreased. Under combined high pCO2 and high-temperature, the increase in CO2 in the surrounding environment may have compensated in part for the decrease in production of CO2 through the host respiration. The opposing observed trends in photosynthesis and respiration rates may show a disruption of the symbiosis.
The lack of a significant effect of CO2 on respiration may result from the near-zero respiration rates found at the higher temperature. The very low respiration rates recorded may be due to the location of the measurements, the body wall, which is not a metabolically active location [20]. Moreover, it was reported that photosynthesis results in buildup of oxygen in the coral tissue [20]. This increased internal oxygen concentration may have rendered the fluxes of oxygen from the surrounding water close to zero or even positive, as it was observed when the respiration rates were the lowest, i.e. under high-temperature stress. A longer adaptation time than the 5 minutes used should be considered to measure accurately low respiration rates by first depleting the internal pool of oxygen. Decreased respiration rates at high-temperatures were also reported for other coral species [37]. In a study with microsensor techniques, a similar but smaller decrease in respiration rates between 29 °C and 32 °C was observed for G. fascicularis [21]. However, some corals also showed an increase or no change in respiration rates under high-temperature stress [38–40]. This discrepancy may be due to the different temperatures used in the experiments. As seen for photosynthesis [40,41], respiration may increase with temperature until a threshold value, above which it decreases. In our case, the temperature of 32 °C may have been overly high and may have caused the threshold value to be reached. A hypothesis to explain the decrease in respiration rates under high-temperature stress is that elevated temperature may have damaged the mitochondrial electron transport system, the enzymatic chain leading to the reduction of oxygen, due for example, to the formation of reactive oxygen species in the mitochondria [42,43]. The increase in CO2 did not affect the coral respiration rates. This result differs from the findings of previous studies that showed an increase in respiration with increased pCO2 [40,44]. More research is still required to understand the impact of acidification on coral respiration.
The low ATP contents observed for corals incubated at 32 °C could be due to the extreme decrease in respiration rates observed or a decrease in the amount of photosynthates translocated. At 32 °C and high pCO2, the net photosynthesis did not decrease as much as under high-temperature stress alone. In this case, the low respiration rates may indicate that the coral hosts could not use the photosynthates supplied through photosynthesis.
Our results are in agreement with previous measurements at the micro-scale, which showed extreme diel variations of pH in the DBL [45]. Under normal pCO2 and between light and dark conditions, the corals experienced a variation in pH of approximately 0.8 pH unit in the DBL. Such variations are greater than –0.3 pH unit, the expected change in pH due to global change over 100 years. These diel variations were amplified by the addition of CO2, with a difference between dark and light of nearly 1.1 pH unit (Fig. 5). The impacts of acidification on corals may vary between day and night. During the day, corals may be able to tolerate the predicted decrease in the seawater pH of 0.3 unit, because the high photosynthetic rates prevent a change in the pH in the microenvironment around corals. However, acidification becomes important during the night and may affect corals. An amplification of diel variations in pH in seawater has been predicted due to the lowering of the buffering capacity of CO2-enriched water [46] and has been confirmed by mesocosm experimentation [47]. Our results show an attenuation of acidification during the day at the micro-scale, due primarily to photosynthetic activity. A similar attenuation was shown to occur at the reef scale due to the presence of macro-algae with a high photosynthesis:respiration ratio in upstream water on coral reefs during the day [15,48]. The pH in the DBL was reduced in the dark by the addition of CO2 in combination with the CO2 produced by respiration. At this low pH, the metabolism of the coral host, energy allocation, may have been more severely affected than during the daytime, when the pH in the DBL was not decreased relative to the control.
Lower ATP contents during the night have been reported for corals and have been attributed to a higher consumption of ATP in the dark compared to under light [11]. In this study, ATP was measured in the morning, after a couple of hours of light period, which may have minimized the effect of acidification on the host ATP contents compared to what it would have been if measured during the night. Even if the effect of an increase of CO2 on host ATP could not be shown at the 95% level of confidence, it is probable that CO2 alone may lead to higher consumption, or lower production, of ATP as a small, but non-significant, decrease of ATP contents at high pCO2 and 27 °C was observed (P = 0.06).
Relatively few studies have investigated the impact of a combined high-temperature and a decrease in the ambient pH on the coral calcification process. The internal pH of corals, especially the pH in the sub-calicoblast space, the site of calcification, needs to be maintained at a high value to precipitate calcium carbonate [10]. Two processes maintain the pH; calcium-ATPase pumps protons from the sub-calicoblast to the coelenteron, and the protons in the coelenteron are titrated by OH− produced through photosynthesis [49]. The decrease in respiration rates and ATP contents of host tissues under high-temperature may have lead to a decrease in the pH at the calcification site due to the lack of energy available to the calcium-ATPase. Moreover, under high-temperature and 400 ppm of CO2, photosynthesis also decreased and may have led to a further decrease of the sub-calicoblastic pH, due to a decrease in the OH− produced. These mechanisms may explain the reported decrease in the calcification of bleached corals [21,50].
Micro-scale studies should be considered for the study of the impacts of stresses on coral physiology. Our results confirmed that water chemistry, including pH, in the microenvironment surrounding benthic organisms is highly influenced by their metabolism and may differ from the chemistry of the surrounding environment. This difference may therefore enhance or mitigate the stress due to environmental changes.
Disclosure of interest
The authors declare that they have no conflicts of interest concerning this article.
Acknowledgments
This work was supported by Grant-in-Aid for Scientific Research on Innovative Areas “Coral reef science for symbiosis and coexistence of human and ecosystem under combined stresses” (No. 20121003) of the Ministry of Education, Culture, Sports, Science and Technology (MEXT), Japan and Global Coral Reef Conservation Project (GCRCP) of Mitsubishi Corporation and the “International Research Hub Project for Climate Change and Coral Reef/Island Dynamics” at the University of the Ryukyus.