1 Introduction
One of the most remarkable features of the retina is its extremely ordered structure. As many central nervous system regions, the vertebrate retina is arranged in a multi-layered assembly. The cell bodies of the five types of neurons are distributed among three cellular layers separated by two synaptic strata where connections are constrained. The inner plexiform layer (IPL) is a multi-layered synaptic neuropil constituted of bipolar cell processes, which synapse on retinal ganglion cell (RGC) dendrites, as well as modulatory connections from amacrine cells. The outer plexiform layer (OPL) is formed by ribbon synapses comprising pre-synaptic horizontal and photoreceptor cells and the post-synaptic bipolar cells [1,2]. In addition to this vertical distribution, within the cellular layers, neurons are non-randomly arranged in regular arrays in the horizontal plane. Cell bodies are evenly distributed and their dendrites tiled. These patterns are called “retinal mosaic”. This very precise organisation is essential in order to establish a functional circuitry of the retina.
Shortly after becoming specified, neurons begin to elaborate neurites and migrate to their final layer. In the mouse, this phase ranges from embryonic day 12 (E12) to post-natal day P7 [3]. All retinal cells derive from a common progenitor, and differentiate following a precise chronological sequence. Ganglion cells, cone photoreceptor, horizontal cells differentiate before birth, while amacrine cells start pre-natally but finish post-natally. Finally, rod photoreceptors, Müller glia and bipolar cells only begin to differentiate after birth [4]. Retinal cells then have to find their way to their final position to cover all the surface of the retina. Then, retinal neurons grow processes and form synaptic contacts within specific layers. Until recently, only a few molecules involved in setting up this complex retinal structure was known. However, genetic tools have led to the discovery of new molecules, which control the different steps that organize the stratification of the retina.
Here, we will review recent data on the mechanisms controlling retinal layering. We will focus on two aspects: the formation of the two plexiform layers and the mosaic patterning.
1.1 Formation of the inner plexiform layer
In mice, the IPL starts to form shortly before birth. In 1893, Ramon y Cajal had already subdivided the IPL into five sublaminae based on his observations of retinal cell processes [5]. In the 1980s, the improvement of neuroanatomical technics allowed for a better description of the IPL and the specificity of each sublamina [6]. Nevertheless, it is only recently that we have begun to understand how bipolar and amacrine cells synapse on the right RGC dendrite to form specific ON/OFF pathways.
Time-lapse studies of the zebrafish retina have shown that RGC dendrites, bipolar cell axons and amacrine neurites seem to grow toward the IPL in a guided manner [7]. Around P14 in mice, the IPL is constituted of more than 10 sublaminae. Structure and function in the IPL are very closely related. For instance, retinal neurons with receptive field centres that are depolarized (ON-cells) by an increase in light intensity have dendrites that project in the innermost sublaminae within the IPL, while hyperpolarized (OFF-cells) will stratified their neurites in the outer sublayers [8]. How do neuronal processes find their appropriate sublamina? In the last five years, numerous molecules have been implicated in the guidance of the IPL processes.
Up to now, five families of molecules were involved in the formation of the IPL, including, several proteins from the immunoglobulin (Ig) superfamily. Sidekick-1 and -2, contain 6 Ig motifs, 13 fibronectin type III (FNIII) repeats and a carboxy-terminal motif (S/TxV) predicted to bind PDZ domains. Sidekicks were discovered through a differential screen of cDNA libraries constructed from single chick RGCs differing by their size and cell surface characteristics. Sidekick-1 and 2 are expressed in the ganglion cell layer just when it starts to be distinguishable from the inner nuclear layer. At E15, Sidekick-1 is expressed in the S4 sublamina of the IPL, while Sidekick-2 is detected in S2 and S4 [9] (Fig. 1). Loss of function experiments has shown that Sidekick-1 and Sidekick-2 are required for lamina specific arborization of processes in the IPL [10]. Overexpression of Sidekick-1 or 2 in the retina redirects neurites to the sublamina in which the corresponding endogenous protein is normally absent [10].
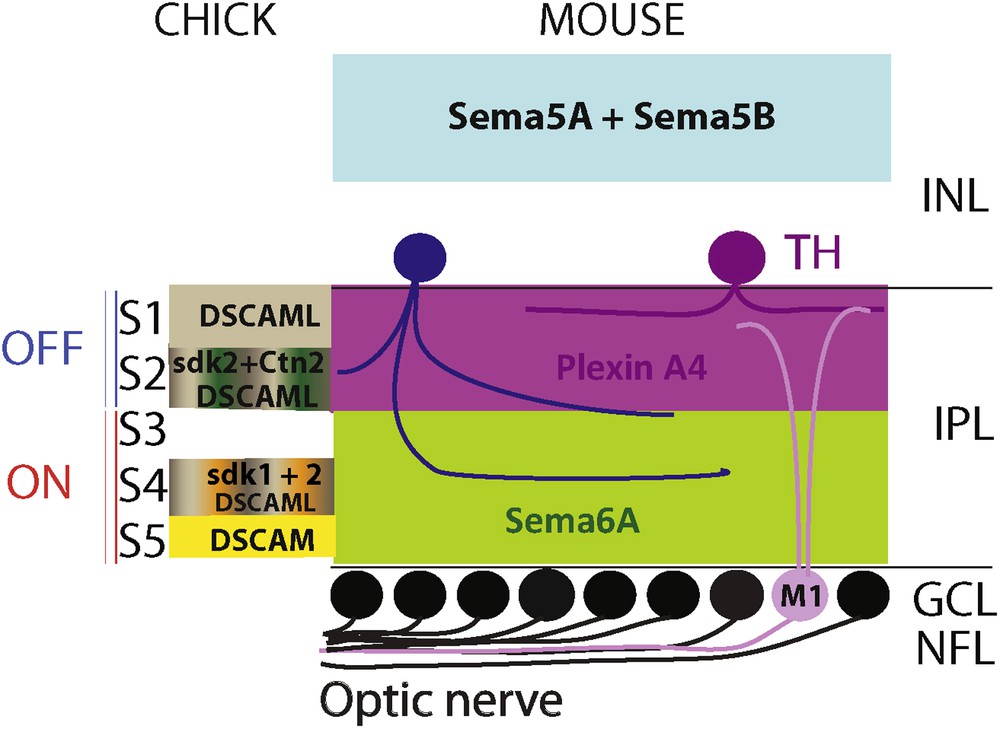
(Colour online) Molecules regulating inner plexiform lamination. On the left, the expression pattern of the different molecules identified for their role in the IPL stratification in the chick. Sidekick-1 is present in S4 while Sidelick-2 is in S2 and S4 together with DSCAML detected also in S1. DSCAM is found only in S5, and Contactin-2 in S2. In mice, Sema5A and Sema5B are present in the outer retina constrain amacrine and bipolar cells neurites toward the IPL. Sema6A-PlexinA4 signalling is necessary for neurite sublamina targeting of amacrine cells and M1-ipRGC.
Adapted from [17].
A close relative of Sidekick, Down Syndrome cell adhesion molecule (DSCAM) is also implicated in IPL stratification. This Ig molecule was already known in Drosophila for its role in axon tiling and dendrite self-avoidance [11,12]. In the chick, two Dscams were cloned, Dscam and DscamL, and just like the Sidekicks, they are expressed by distinct subclasses of retinal neurons [10]. More precisely, in the chick IPL, Dscam is expressed in S5 and DscamL in S1, S2 and S4 (Fig. 1). Loss of function of Dscam using interfering RNAs results in the development of ectopic R-cadherin positive processes in S4 instead of S5. Conversely, when Dscam is overexpressed, all the neurites are condensed in S5. Similarly, processes overexpressing DscamL do not project in S3, which is normally DscamL-negative. Thus, at least in the chick, it is likely that these two proteins together with the sidekicks regulate the stratification of the IPL. Indeed, inDscam–/− mice retinal ganglion cells dendrites are fasciculated and cell bodies are clustered. Similarly, bipolar cell processes are also bundled and AII amacrine soma clumped in Dscaml1–/− knockout mice suggesting that these molecules have a role in self-avoidance rather than in lamina specification [13].
As mentioned above, the C-termini of Sideckiks and DSCAM present a motif predicted to bind PDZ domains. Using Sidekick-2, Yamaga and Sanes identified a family of binding partners: the membrane-associated guanylate kinase with inverted orientation (MAGI) [14]. MAGI-2 is co-expressed with sidekick-2 in S2 and S4 in the IPL. Moreover, when MAGI-2 is knocked down by in vivo electroporation of retroviruses expressing RNAi specific for MAGI-2, Sidekick-2 expression becomes diffuse in the IPL, and Calbindin-positive processes are not restricted anymore to their sublaminas (S2, S3, S4). Therefore, it is likely that Sidekick-2 function requires its interaction with MAGI-2.
Another family of Ig molecules, the contactins (Cntns) have been identified for their role in chick IPL formation. Just like the Sidekicks, they have 6 Ig domains, but only 4 FnIII domains and are anchored to the membrane by a glycosylphosphatidylinositol (GPI) moiety. DNA sequence analysis indicates that Dscams, Sidekicks and Cntns are close relatives [15]. In the chick retina, all five Cntns (Cntn1-5) are expressed in distinct sublaminas of the IPL. For instance, Cntn2 is expressed in S2 and S4 (Fig. 1). When Cntn2 expression is depleted using RNAi, Sidekick-1 expression in the IPL is more diffused instead of being restricted to S4, but Sidekick-2 expression is not perturbed. Conversely, when Cntn2 is overexpressed, all the neurites are confined to S2 and S4. Therefore, it is likely that Cntns control the stratification of processes in the IPL. How do Cntns proceed to guide the processes to the appropriate sublamina? The most likely hypothesis is that they act by homophilic adhesion. When K562 cells that do not express any known adhesion molecules, are transfected with Cntn2, they aggregate, whereas untransfected cells do not [15]. Cntn4, like Cntn2, is also able to aggregate cells, but not Cntn1, 3 and 5. In conclusion, it is likely that the 3 related families, Sidekicks, Dscams and Cntnsplay play an important role in the guidance of retinal neurites to their appropriate IPL sublamina.
More recently, members of the semaphorin family of axon guidance molecules, together with their receptors the plexins, were shown to regulate stratification of the IPL. First, in PlexinA4 mutant mice, as well as in Semaphorin6A-deficient mice, the IPL stratification is totally aberrant [16]. More precisely, dopaminergic amacrine cells processes, which project in S1 in wild-type mice, stratify in all the IPL of the mutant mice. Likewise, M1-ipRGCs (M1 type of intrinsically photosensitive retinal ganglion cells) that normally stratify in S1 also send aberrant neurites to other sublaminas. PlexinA4 is expressed in S1 and S4 and in dopaminergic amacrine cells, but not in M1-ipRGCs. On the other hand, Semaphorin6A is present in S3 to S5 in the IPL. More precisely, PlexinA4 is expressed in the OFF sublayers, while Sema6A is in the ON sublayers (Fig. 1). Thus, it is likely that Semaphorin6A acts as a repulsive ligand for PlexinA4 to control dopaminergic amacrine cells targeting to the IPL. Concerning M1-IpRGCs, they could use cues provided by dopaminergic amacrine cells to target the proper sublamina. This study provides evidence that Sema6A-PlexinA4 signalling is necessary for neurite sublamina targeting, but do not initially guide the processes to the IPL. In another study, two other semaphorins, Sema5A and Sema5B, were shown to coerce processes from bipolar, amacrine and retinal ganglion cells to the IPL. During IPL development, Sema5A and Sema5B, which are present in the outer retina, prevent any retinal processes to develop toward the outer retina (Fig. 1). This is mediated by the PlexinA1 and PlexinA3 receptors [17]. A role for semaphorins in lamina specification has also been described in other regions of the nervous system [18–20].
Finally, an unexpected molecule has been shown to regulate the stratification of the IPL. The phosphatidylinositol (3,4,5)-trisphosphate [PtdIns(3,4,5)P3] phosphatase and tensin homolog (PTEN) is a tumour suppressor gene, which codes for a lipid phosphatase [21]. First, it was found to regulate retinal ganglion cell terminal arborisation in the Xenopus [22]. PTEN protein is present at a high level in the mouse IPL during the phase of dendritic development and synaptogenesis [23]. In PTEN-knockout mice, the IPL is broader and the sublaminas stratification is totally disrupted. These morphological defects are associated with deficits in visual function.
1.2 Formation of the outer plexiform layer
The outer plexiform layer is the less complex of the two synaptic plexuses in the vertebrate retina, but its development has not been studied extensively. The formation of the OPL occurs post-natally. After differentiation, horizontal cells migrate to the outer part of the INL, establishing the forthcoming location of the OPL. In the meantime, at least in the ferret, photoreceptor cells spread neurites toward the IPL. This is followed around P4 in mice by horizontal cells, which will first develop dendritic terminals that will contact the pedicles of cone photoreceptor cells. They will also extend an axon, which will connect with the spherules of rod photoreceptor cells [24,25]. Then, starting at P10, the post-synaptic elements will invaginate in order to form a pre-synaptic ribbon.
Horizontal and bipolar cells require photoreceptor cells to form synapse in the OPL. In mutant mice lacking photoreceptor cells, horizontal cells sprout in the ONL, instead of the OPL, and bipolar cells processes grow along the horizontal cells neurites [26]. In order to synapse in the OPL, horizontal cells also need to reach the outer part of the inner nuclear layer. In the Lim1–/− mice, horizontal cells remain in the inner part of the INL and project their neurites within the IPL rather than the OPL [27].
Not much is known about the molecular mechanisms that control neurite targeting in the OPL. During light deprivation, rods and cones cells release glutamate continuously. When they are stimulated by light, photoreceptors are hyperpolarized which lower Ca2+ influx and decrease glutamate release. Thus, it is not surprising that many of the molecules that were found to regulate synapse formation of the OPL belong to the Ca2+ pathway. For instance, Cacna1f, which is a subunit of the Cav1.4 calcium channel, is involved in ribbon synapse formation in the OPL. In Cacna1f mutant mice, the presence of synaptic molecules, such as bassoon and mGLUR6 is greatly reduced in the OPL compared to wild-type mice. Moreover, the OPL is disrupted and ectopic processes coming from rod bipolar and horizontal cells are detected in the outer nuclear [28,29]. Another subunit of a calcium channel has also been implicated in the OPL formation: the L-type calcium channel auxiliary subunit of the alpha2delta type coded by the Cacna2d4 gene. In this spontaneous mutant, the photoreceptor ribbon synapses are affected and the width of the OPL is considerably reduced. Nevertheless, it cannot be excluded that this phenotype is a consequence of a significant loss of rods [30]. Giving the importance of calcium channels for photoreceptors ribbon synapse formation, it is somehow expected that molecules regulating these channels are also essential for the development of the OPL. For instance, the loss of the calcium binding protein, CaBP4, which modulates voltage gating of Cav1.4, results in a reduction of the number of photoreceptors ribbon synapses [31].
Some studies strongly suggest that the pre-synaptic protein, Bassoon, is necessary for the formation of photoreceptor ribbon synapse in the OPL, by controlling the attachment of the ribbon to the active zone [32].
More recently, it has been shown that Sema6A and its receptor PlexinA4 are involved in OPL development. Sema6A–PlexinA4 signalling controls horizontal processes targeting to the OPL and PlexinA4 guides the horizontal cell axon to the rod ribbon [33]. New genetic tools to label pre- or post-synaptic partners in the OPL might help in improving our understanding of its development [34,35].
2 Mosaic patterning
Within their layers, photoreceptor, horizontal, amacrine and retinal ganglion cells are arranged in a non-random array across the retinal surface often referred to as mosaic patterning. It is likely that this horizontal organisation is needed to cover the entire retina and to avoid blind spots, as a random distribution would lead to local alterations of the visual space. Moreover, the more complex the colour system, the more precise the mosaic [36].
Mosaic patterning has been thoroughly described for cones, horizontal cells, ganglion cells and for some types of amacrine cells which all differentiate pre-natally [3]. Although, it cannot be ruled out that bipolar cells and rods are not arranged in a regular array, this might just reflect the lack of appropriate cellular markers to study such organisation.
The cone mosaic has been mostly described in higher vertebrates, which have a more complex colour system than the mouse where 97% of the photoreceptors are rods [36–39]. Nevertheless, cones in the mouse retina are also organised in a mosaic. A transgenic mouse expressing GFP under the control of human red/green opsin gene 5′ sequences was generated to analyse how the cone mosaic develops. The analysis of the movement of cone cells by time-lapse imaging showed that the development of cone patterning occurs in two steps [40]. First, between E18 and P10, differentiating cones directly form a non-random array. Then, while the cone cells are maturing (P10–P20), the regularity of mosaic pattern is gradually refined, probably by tangential dispersion, until cone synaptic contacts are made [41].
The mosaic patterning of horizontal cells has been more studied, maybe because they are less numerous than the other cell types and organized in a single layer. In the mouse, the array of horizontal cells begins to form post-natally [42]. In the mammalian retina, horizontal cell bodies are arranged in a regular mosaic and their dendrites send processes radially within the outer plexiform layer, where their unique afferents come from cone photoreceptors. Mature horizontal cells show some overlap of their dendritic fields [43].
There are around 30 subtypes of mammalian amacrine cells [1,44] and their development is correlated to their final position in the inner nuclear layer [45]. The arrays of all the different subtypes have not been studied in details. Nevertheless, for those who have been analysed, it appears that each subtype of amacrine cells forms its own mosaic patterning. For instance, cholinergic amacrine cells, once differentiated, move tangentially away from their birth column to set in a mosaic pattern [46].
Mammalian retinal ganglion cells are also arrayed in a mosaic [36], which is specific for each subtype of RGCs. In the adult cat, ON- and OFF-α-RGCs cell bodies are always paired, close to each other [47]. These pairs are distributed in a regular array in the mature retina [48]. Their dendritic arborisations are also orderly arranged: they cover the whole surface of the retina without overlapping [49,50]. In the mouse, it was recently described that the dendritic arbours of the two subtypes M1 and M2 melanopsin-expressing ganglion cells are monostratified in two different layers in the IPL [51].
The tiling of bipolar cells has been less studied and there is no clear evidence for a mosaic patterning of bipolar cell bodies. Yet, it seems that their dendritic trees tile the retina. For instance, in the mouse, the dendritic arbours and axon terminals of Type 7 bipolar cells are distributed without much overlap [52]. A similar organisation has been observed for rod bipolar axon terminals and calbindin-positive bipolar cells in the rabbit [53,54]. Nevertheless, as bipolar cells are the last retinal cells to differentiate [55], this organized distribution could be a consequence of the already non-randomly array of their synaptic partners.
How do these mosaics form? Several mechanisms have been proposed, and it seems that each mosaic combines some of them (Fig. 2).
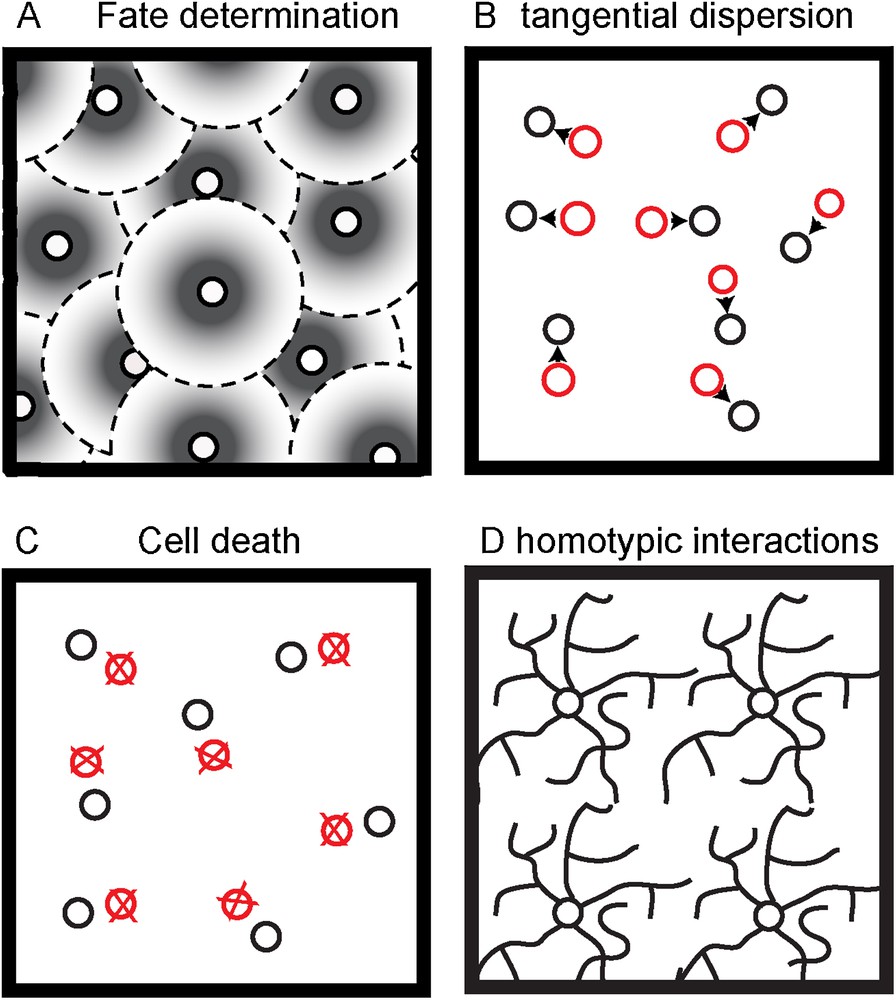
(Colour online) Possible non-exclusive biological mechanisms to create regular cell spacing. A. Fate determination induce adjacent like-type cell to express the same phenotype. B. Adjacent like-type cell repel each other in order to migrate tangentially and cover evenly the surface. C. Over-generated like-type cells to close to each other are eliminated by cell death. D. Dendrites from like-type cells recognize each other in order to avoid overlapping.
Adapted from [82].
First, tiling could be linked to fate determination, as is the case for Drosophila R8 photoreceptor cells, which prevent neighboring cells from taking the R8 fate [56]. In primates, it seems that the first cone photoreceptor could induce adjacent undifferentiated cones to express a particular opsin phenotype. Thus, this process would generate a “protomap” for the development of the cone mosaic [38]. A similar mechanism has been proposed for goldfish cone photoreceptors and dopaminergic amacrine cells [57,58]. In cultures of chick RGCs, less ganglion cells are produced when young RGCs are cultured in conditioned medium from old RGCs. This could suggest that mature RGCs secrete a factor inhibiting RGC development [59]. Accordingly, retinal ganglion cells are distributed in a mosaic early in development, long before they migrate to the ganglion cell layer [60]. Using computer modelling, Eglen and Willshaw investigated whether cell fate determination can occur in the retina [61]. They found that cell fate mechanism could generate a non-random array from undifferentiated cells. Nevertheless, it appeared that cell fate alone is unlikely to generate a mosaic of the same uniformity as observed experimentally. Thus, cell fate is only one of the mechanisms involved in generating the right density of each retinal cell type. One of the reasons is that it occurs very early in development and therefore the following developmental mechanisms might interfere in the regularity of the mosaic. Therefore, other processes might be needed to maintain the regularity. Last, this model does not apply to all retinal cell types as for example, cholinergic amacrine cells do not show any minimal spacing limitation as their migrate to their final layer [46].
The analysis of clones of different retinal cell lineages using transgenic mice expressing ß-galactosidase only in a few progenitors showed that retinal cells could disperse tangentially once they are differentiated [41,62]. Newborn cells can migrate away from their birthplace at distances ranging from 45 to 150 μm, [62]. Dispersed amacrine, horizontal, cone and ganglion cells can be observed from their original column in the same plane. In the rat retina, it was also found that RGCs and cholinergic amacrine cells migrate tangentially but not further than 30 μm away [46]. Likewise, a tangential dispersion of various bipolar and amacrine cells types was also described at early post-natal days in the mouse [63]. However, only calretinin-positive, CD15-positive, Islet1-positive amacrine cells were found to migrate at a significant distance from their original column whereas ON-bipolar cells do not disperse tangentially. The strongest support for the existence of tangential dispersion of retinal cells comes from live imaging studies in mice and zebrafish. In both species, lateral migration of horizontal cells have been observed [42,64].
Cell death is a key regulator of the number of neurons in the nervous system during development. A controlled balance between proliferation and death is a known process to fix the size and the cytoarchitecture of neuronal structures [65,66]. Accordingly, cell death was shown to play a role in the development of retinal mosaics. Computer simulations first showed that during post-natal development, the normal loss of ON- and OFF-α-RGCs transform a random distribution to a regular array [48], but also that cell death is not sufficient to form the mosaic of ON- and OFF-α-RGCs [61]. In transgenic mice overexpressing the anti-apoptotic gene bcl-2, it was observed that dopaminergic amacrine cells were eliminated when they were too close, therefore, creating an exclusion zone [67]. This was later confirmed by the analysis of mice deleted for Baxa proapoptotic factor [68]. The same process occurs for cholinergic cells, which used ATP-induced cell death to discard cells that are too close to one another [69]. Recently, it was shown that the mosaic patterning of ipRGCs requires apoptosis [70]. In Bax-knockout mice, in which RGCs do not undergo apoptosis, ipRGCs are clustered instead of being evenly distributed. However, cell death is not a mechanism involved in the patterning of all retinal mosaics. For instance, the mosaic organisation of horizontal cells probably relies on another mechanism. Indeed, there is no direct evidence that they undergo naturally occurring cell death during and after their differentiation [71]. Moreover, there is no evidence for an overproduction of horizontal cells during development.
The last mechanism that is likely to participate in mosaic patterning is the interactions between homotypic cells. The first experimental argument came from the work of Perry and Linden who removed subsets of ganglion cells from post-natal rat retina and therefore could evaluate the role of interactions between neighbouring cells later in development [72]. They observed that the dendrites from cells near the depleted area preferentially extend their arbour toward the empty region. Therefore, ganglion cell developing dendrites do not grow randomly but fill preferentially vacant regions. This study was the first observation that led to the concept of “tiling”. This notion refers to the capacity of dendrites from individual neurons of the same neuronal type to cover the sensory field as much as possible but with minimal overlap.
Cell avoidance was first observed in the leech where sister branches from the same neuron do not contact each other but overlap with neurites from different cells [73] (Fig. 2). In the rat retina, when microtubules are perturbed within dendrites, the monolayered retinal arrays fail to organize with regular spacing [74]. The mosaic of horizontal cells is not perturbed in coneless mice [75–77], which suggests that this non-random array is not induced by photoreceptor cell afferents, but more likely involves homotypic interactions between horizontal cells. Moreover, the primary dendrites of horizontal cells develop before there are contacted by photoreceptors. However, the formation of higher order branches requires photoreceptors contacts [77].
Which are the molecules mediating mosaic patterning? In the chick retina, N-cadherin was first shown to control the size of the dendritic field of horizontal cells through the regulation of the attachment of their dendritic processes to photoreceptor cells [78]. More recently, cadherin-like proteins were shown to be required for self-avoidance of starburst amacrine cells [79]. As for plexiform layers, Ig molecules are involved in mosaic patterning and in particular, the DSCAMs. In Dscam knockout mice, tiling and self-avoidance is perturbed in melanopsin-retinal ganglion cells, dopaminergic and bNOS (neuronal nitric oxide synthase)-positive amacrine cells triggering fasciculation of their neurites [68,80]. Interestingly, the other types of amacrine cells are not affected. In Dscaml1 knockout mice, rod bipolar cell dendrites are fasciculated and aII amacrine cell bodies are clumped, suggesting that DSCAML1 plays a role in self-avoidance [13]. Recently, a screen for molecules specific of different retinal cell types, led to the identification of two new transmembrane proteins, MEGF10 and MEGF11 [81], controlling the mosaic pattering of starburst amacrine cells and horizontal cells. More surprisingly, ionotropic glutamate receptors seem to be able to cause tangential dispersion retinal cells from their birth column [63].
3 Conclusions
Decades of research has led to a deeper understanding of how retinal neurons find their way to their final destination and arborize in their appropriate synaptic layers. Although this review was focused on vertebrates, one has to keep in mind that, vertebrates and invertebrates visual circuits share design principles and molecules, and that what we learned from the Drosophila eye helped in understanding the development of retinal stratification. It is clear that only a few of the mechanisms involved in the development of retinal layers have been identified, and that our knowledge remains partial. Nonetheless, new genetic tools, animal models and imaging technics should help unravel the different steps implicated in the layering of the retina.
Disclosure of interest
The authors declare that they have no conflicts of interest concerning this article.