1 Introduction
Heavy metals released from metalliferous mining and use of chemicals in agricultural practices can generate damaging effects on various agro-ecosystems including plants [1], animals, and humans via the food chain [2].
Heavy-metal toxicity to some plants and microorganisms is well documented, but its effect on legumes, Rhizobium and legume–Rhizobium symbiosis is less understood [3]. These metals can arrest the growth and multiplication of Rhizobia in the rhizosphere and may also have a depressive effect on the process involved in legume–Rhizobium symbiosis, resulting in low nitrogen fixation [4]. However, microorganisms exposed to the higher concentration of toxic heavy metals have evolved various methods to resist metal stress [5]. One of the microbial processes as a bioremediation tool to remove heavy metals from soils is bioaccumulation [6], which is an active method dependent upon the metabolic energy of microorganisms [7]. Metals can also cause severe toxicity to the metabolic activities of various legumes including photosynthesis, synthesis of proteins, of enzymes, and of carbohydrates.
Therefore, understanding the metal–rhizobia–legume interaction in a metal-enriched environment is urgently required for growing legumes in soils contaminated with heavy metals [8]. Recently, an alternative strategy has been developed that provides plants with certain beneficial soil microorganisms [9]. This remediation process, known as rhizoremediation, utilizes plant–microbe interactions to improve the efficiency of phytoremediation. The inoculation of genetically modified, cadmium-resistant bacteria (Pseudomonas putida) enhanced by 40% cadmium accumulation in sunflower plants (H. annuus) compared to the control group [10]. Additionally, a few naturally occurring microorganisms with high metal resistance in plant rhizosphere significantly increased metal uptake in plants and reduced metal toxicity [11,12]. Some metal-resistant microorganisms, such as plant-growth promoting bacteria (PGPB), are able to produce plant hormones and siderophores in addition to solubilizing phosphate, which reduces metal stress and promotes plant growth, resulting in improved total metal uptake by plants [13,14].
Copper is an essential trace element, serving as a cofactor for a variety of enzymes such as ascorbic acid oxidase, polyphenol oxidase and superoxide dismutase [15]; therefore, an optimum copper concentration ensures a normal growth and development of plants [16]. However, excess Cu inhibits plant growth and impairs important cellular processes. The toxicity of Cu is associated with a series of visible symptoms, including chlorosis and necrosis of the leaves, short stems, browning of the root, and abnormal root morphology. Visible symptoms of metal toxicity stress in plants are indications of abnormalities at the structural and ultrastructural levels [17].
Thus, the development of phytoremediation strategies like the interactions in the rhizosphere of plants growing in contaminated soils are important because of the biotechnological potential of microorganisms for metal removal directly from soil or the possible transference of them to the plants [18]. Copper soil contamination leads to oxidative stress generation in plants, due to an increase in the levels of reactive oxygen species (ROS) within sub-cellular compartments. To overcome this, cells are equipped with enzymatic mechanisms to eliminate or reduce their damaging effects. The antioxidant enzymes such as superoxide dismutase (SOD), catalase (CAT), peroxidase (POX), and ascorbate peroxidase (APX) have a vital role in scavenging ROS and thereby in preventing oxidative damage. The balance between ROS generation and eradication determines the survival of the system [19,20].
In this work, Rhizobium and PGPR were selected to be used as inoculants for Vicia faba legume under Cu contamination in hydroponic conditions. Our purposes were (i) to isolate and characterize copper-resistant bacteria from root nodules of Vicia faba growing in different mining and agriculture regions, (ii) to evaluate the ability of the inoculums for Vicia faba growth promotion in the presence of different Cu concentrations, (iii) to assess the effect of inoculums on copper accumulation in Vicia faba organs, and (iv) to study the modification of antioxidant enzymes activity in order to determine the mechanism involved in copper tolerance.
Thus, this experiment may provide strains tolerant to copper stress that can be used for Vicia faba inoculation to enhance natural detoxification of copper-contaminated environments.
2 Materials and methods
2.1 Isolation of strains from contaminated soils
Composite surface soil samples from the top 15 cm were collected from mining and agricultural fields of Tunisia, and bacteria were isolated by nodulation using Vicia faba legume [21,22]. Seeds were germinated and grown on soils from the contaminated site, placed in a controlled plant growth chamber and watered with Hoagland nutrient solution without nitrate. After 36 days, nodules that had formed on plant roots were cut off and bacteria isolated from them as follows: nodules were surface sterilized with 70% ethanol for 5 min and exhaustively washed in sterile distilled water. Afterwards, they were crushed and bacteroids from the nodule were isolated on Yeast Extract-Mannitol (YEM) agar plates [23].
2.2 Determination of copper minimum inhibitory concentration (MIC) values of used strains
The minimum inhibitory concentration (MIC) of copper was tested on agar plates containing either YEM or Tryptone–Yeast extract (TY) media [24], with increasing concentrations of copper. Maximal concentrations used for resistance tests were similar to the copper concentration found in contaminated soils. Cu (in the form of copper sulfate CuSO4) was used at concentrations of 0, 0.2, 0.5, 1 and 2 mM. Then, plates were incubated at 28 °C for 48–72 h. Four selected strains (SF7, SF11, SF28 and SF29) growing in a primary screening test were inoculated into 50 mL YEM medium supplemented with 0, 0.3, 0.5, 1 and 2 mM Cu2+, respectively, and were incubated at 28 °C with shaking at 150 rpm for 3 days. Growth was monitored at 12 h intervals as the optical density of 600 nm (OD600) with a PerkinElmer UV/VIS spectrophotometer.
2.3 Bioaccumulation of copper in selected strains
Cells (0.1 g dry weight) of the four selected strains were inoculated into 100 mL of a TY medium containing (0.5, 1 and 2 mM Cu) and incubated at 28 °C with agitation at 150 rpm. Cells were harvested 12 and 24 after inoculation by centrifugation at 6000 g for 25 min. After washing three times with sterilized double-distilled water, the cell pellets were agitated with 10 mM of sterilized EDTA at 28 °C under stirring at 150 rpm for 10 min in order to remove the copper ions adsorbed to the cell's surface. After further centrifugation at 14,000 g for 25 min, the cells were suspended in 5 mL of 0.1 M HNO3, and the solution was centrifuged at 14,000 g for 30 min. The supernatant was used for the determination of cell wall-bound Cu2+ [25] with Atomic Absorption Spectrometry (AAS), respectively.
After determination of dry weights, the cell pellets were digested with H2SO4–HClO4 (v:v = 3:1) at 110 °C for 3 h and diluted with double distilled water for the determination of intracellular accumulated Cu2+ [26].
2.4 DNA preparation, primer design and PCR
Regarding DNA extraction, the method described by [27] was used. For amplifying 16S rRNA genes, primers (27f, 5’ GAGAGTTTGATCCTGGCTCAG and 1495r, 5’CTACGGCTACCTTGTTACGA) were used and amplified near the 1350 base pairs region using Bio-RAD Thermal Cycler model (C1000).
A 25-μl reaction mixture including (10 to 50 ng) of bacteria DNA template, 2.5 mM 10X buffer, 1.5 U taq polymerase, 1.5 mM MgCl2, 200 μM dNTP, and 1 μM of each of the primers was used. The reaction conditions were: initial denaturizing of 15 min at 94 °C, which was followed by 34 cycles of denaturizing of at 94 °C (1 min), annealing of at 55 °C (1 min) and extension at 72 °C (1 min). Final extension has also been done at 72 °C during 10 min.
2.5 Sequencing and phylogenetic analysis of the 16S rRNA gene
The 16S rRNA gene of the representative strains for each combined cluster was amplified with the same primers and the same procedure as in the restriction analysis, and was sequenced directly by using a PCR-based method. GenBank BLAST (N) was used for homology searches. Phylogenetic and molecular evolutionary analyses were conducted using MEGA version 4.1(30). Nucleotide sequence similarity searches were conducted by Genbank BLAST (N). The ribosomal RNA gene sequences were submitted to the GenBank database.
2.6 Plant materials and hydroponic experiments
Seeds of Vicia faba, commercial variety (cv. Bachaar), were obtained from UTAP (Union tunisienne de l’agriculture et de la pêche, Tunisia), were sterilized in hypochlorite at 5% for 2 min. The seeds were washed with distilled water and redistilled water, then sown in vermiculite in plastic pots. Four days after germination, the seedlings were used in the experiments. Uniform seedlings were transferred to 160 hydroponic pots, each one containing 500 ml of a modified Hoagland solution [28]. Plants were cultivated in a controlled-environment growth chamber at 25 °C with a 16:8 h light: dark photoperiod with a 70% relative humidity. After 7 days of growth in hydroponic culture, 80 plants were inoculated with a consortium of bacterial strains identified as Rhizobium sp. CCNWSX0481, Rhizobium leguminosarum bv. viciae, Enterobacter clocae and Pseudomonas sp. These four strains were selected among 30 isolates from Vicia faba nodules for efficiency and copper tolerance (Table 2).
Intrinsic resistance to copper of isolates.
Strains | Nodulation Test |
Nodules Plant |
MIC Cu (mM) |
SV1 | – | 0 | 0.25 |
SV2 | + | 4 | 0.25 |
SV3 | + | 12 | 0.15 |
SV4 | − | 0 | 0.05 |
SV5 | + | 16 | 0.2 |
SV6 | − | 0 | 0.15 |
SV7 | − | 0 | 0.15 |
SV8 | − | 0 | 0.15 |
SV9 | + | 12 | 0.2 |
SV10 | + | 16 | 0.2 |
SV11 | + | 15 | 0.2 |
SV12 | + | 24 | 0.2 |
SV13 | + | 8 | 0.25 |
SV14 | + | 14 | 0.25 |
SV15 | + | 22 | 0.5 |
SV16 | − | 0 | 0.35 |
SV17 | + | 9 | 0.3 |
SV18 | − | 0 | 0.35 |
SV19 | − | 0 | 0.35 |
SV20 | + | 16 | 0.5 |
SV21 | + | 9 | 0.35 |
SV22 | + | 12 | 0.35 |
SV23 | − | 0 | 1 |
SV24 | + | 6 | 0.25 |
SV25 | − | 0 | 0.35 |
SV26 | + | 14 | 0.35 |
SV27 | − | 0 | 2 |
SV28 | − | 0 | 0.3 |
SV29 | − | 0 | 0.35 |
SV30 | − | 0 | 0.35 |
Strains were grown in an Erlenmeyer flask containing a YEM (yeast extract manitol) medium on a rotating incubator under continuous stirring at 200 rpm for 3–5 days at 28 °C. For the co-inoculation treatment, equal amounts of bacterial inoculums were mixed. After 7 days of inoculation, three types of treatments were used: 0.5, 1 and 2 mM supplied as CuSO4 for 14 days. Shoot and root dry matters were determined after drying the washed freshly seedlings in an aerated oven at 80 °C to constant weight (Table 1).
Experimental dispositive of hydroponic culture of Vicia faba co-inoculated with bacterial consortium in presence of copper treatment.
Control Plants (TO) | Plants (TO) + inoculation | Plants (T0) + Cu treatment | Plants (T0) + Inoculation + Cu | ||
Germination | Time | 6 days (4 in the dark and 2 under light) | 6 days (4 in the dark and 2 under light) | 6 days (4 in the dark and 2 under light) | 6 days (4 in the dark and 2 under light) |
Medium | Agar 9% | Agar 9% | Agar 9% | Agar 9% | |
Conditions | 24 °C | 24 °C | 24 °C | 24 °C | |
Preculture | Time | 6 days | 6 days | 6 days | 6 days |
Medium | Hoagland medium | Hoagland medium | Hoagland medium | Hoagland medium | |
Conditions | Perlite sterile | Perlite sterile | Perlite sterile | Perlite sterile | |
25 °C (16 h/8 h; day/night) | 25 °C (16 h/8 h; day/night) | 25 °C (16 h/8 h; day/night) | 25 °C (16 h/8 h; day/night) | ||
Inoculation | Conditions | Co-inoculation in preculture step | Co-inoculation in preculture step | ||
Rhizobium leguminosarum bv. viciae | Rhizobium leguminosarum bv. viciae | ||||
Without inoculation | Rhizobium | Without inoculation | Rhizobium | ||
Pseudomonas | Pseudomonas | ||||
Enterobacter cloacae | Enterobacter cloacae | ||||
Hydroponic culture | Time | 45 days | 45 days | 45 days | 45 days |
Medium | Hoagland medium + Azote | Hoagland medium | Hoagland medium + Azote | Hoagland medium | |
1 plant/pot of 1 L (20 pots) | 1 plant/pot of 1 L (20 pots) | 1 plant/pot of 1 L (20 pots) | 1 plant/pot of 1 L (20 pots) | ||
Conditions | Medium changement every 7 days | Medium changement every 7 days | Medium changement every 7 days | Medium changement every 7 days | |
Copper treatment | Time | 15 days (after 30 days of culture) | 15 days (after 30 days of culture) | ||
Medium | Without copper treatment | Without copper treatment | Hoagland medium + CuSO4 | Hoagland medium + CuSO4 | |
Conditions | 0.5 mM Cu (20 pots) | 0.5 mM Cu (20 pots) | |||
1 mM Cu (20 pots) | 1 mM Cu (20 pots) | ||||
2 mM Cu (20 pots) | 2 mM Cu (20 pots) | ||||
Screening | 35th day (5 pots for enzymatic activities) | ||||
Harvest (45 days) |
2.7 Cu content analysis in Vicia faba organs and in nutrient solution
After different treatments exposure, Vicia faba plants were harvested at the age of 45 days and separated into root and leave samples. Roots were rapidly washed in distilled water and the Cu bound to the rhizoderm was removed by HCl followed by rinsing with distilled water [29]. Three samples of 0.1 g of shoot or root tissue were triturated, homogenized with mortar and pestle then used for the determination of copper accumulation. The dried tissues were digested in concentrated HNO3 for 2 h at about 200 °C, and further digested with concentrated HNO3/HClO4 (3/1, v/v) at about 200 °C. After cooling, the extract was diluted with 1 M HNO3 and made up to 10 mL. The Cu content of the extract was determined using a flame atomic absorption spectrophotometer. To determine the uptake of copper ions from the nutrient medium, four milliliters were taken from a Hoagland solution containing 0.5, 1 and 2 mM of CuSO4. The level of Cu2+ in the sample was measured with an inductively coupled plasma–mass spectrometer (ICP–MS) method [30].
The translocation factor (TF) factor for metal within a plant was expressed by the ratio of metal (shoot)/metal (root) to show metal translocation properties from roots to shoots [31].
The following formula was used for calculations: bioaccumulation coefficient = copper concentration in dry plant part (μgmetal·gdry weight−1 of plant part)/copper concentration in nutrient solution (μgmetal·mlsolution−1) [32].
The results presented are the values ± standard error obtained from at least three replicates. Significant differences between treated and control plants are determined using the ANOVA test (P < 0.05).
2.8 Biochemical analyses
2.8.1 Estimation of lipid peroxidation
Lipid peroxidation was estimated using MDA content evaluation on the 45th day of the experiment [33]. In this case, 1 g of fresh tissue was homogenized in 10% (w/v) TCA (10 ml) and centrifuged at 10,000 rpm for 10 min. An equal volume of 10% trichloroacetic acid (TCA) solution containing 0.5% 2-thiobarbituric acid (TBA) and 1% polyvinylpyrrolidone (PVP) was added to the supernatant [34]. The sample was incubated at 95 °C for 30 min, cooled quickly in an ice-bath and centrifuged at 10,000 rpm for 15 min. The absorbance was measured at 532 nm and corrected for nonspecific absorbance at 600 nm. The concentration of MDA was calculated using an extinction coefficient of 155 mM−1·cm−1 as μM (MDA)·g−1 FW.
2.8.2 Enzyme extractions
Fresh root and leaf samples of 1 g were ground in liquid nitrogen using a mortar and a pestle. The ground samples were homogenized on ice in 10 ml of a solution containing 50 mmol·l−1 of potassium phosphate buffer and 1% (w/v) (PVP) (pH 7.8) and kept at 4 °C for 10 min. The homogenates were filtered and centrifuged at 4000 g for 15 min at 4 °C. The supernatant was then assessed for antioxidant enzyme activity. Each enzyme activity was measured against a protein extract-free blank. For POX enzyme, extracts were prepared by mixing 250 mg of plant ground material with 1.2 ml of 50 mM Tris-HCl buffer (pH 7.3) containing 0.5 M of CaCl2 and 5 mM of β-mercapto-ethanol, at 4 °C for 1 h. The homogenate was centrifuged (14,000 g, 4 °C, 45 min) and the resulting supernatant was used as a crude extract of POX. The protein content was determined according to [35], using bovine serum albumin (BSA, Sigma) as a standard.
For antioxidant enzymatic activities determinations on nodules, 50 mg of nodules samples were homogenized in 5 ml of a 100 mM phosphate buffer (pH 7.0) containing 1 mM EDTA 50 mg PVPP with a pestle and a mortar. The homogenate was filtered and centrifuged at 15,000 g for 15 min at 4 °C. The supernatant was collected and assayed for antioxidant enzyme activities.
Superoxide dismutase assay: Superoxide dismutase (SOD) (EC 1.15.1.1) activity was measured using the published method for the photoreduction of nitroblue tetrazolium chloride (NBT) with some modifications. The composition of the reaction mixture (total volume: 3 ml, pH: 8.0) was as follows: 50 mM sodium phosphate buffer (pH 7.8), 0.1 M tris-HCl, 14 mM methionine, 1.05 mM riboflavin, 0.03% TritonX-100, 50 mM nitroblue tetrazolium chloride (NBT), 100 mM EDTA, and 20 μl enzyme extracts. Riboflavin was added last, and the glass test tubes were shaken and placed under fluorescent lamps. The reaction took place during 5 min and was then stopped by switching off the light. The absorbance was measured at 560 nm. Blanks and controls were run in the same manner, but without illumination and enzyme extract, respectively. Reduction of NBT under illumination was measured with or without the addition of the enzyme extract, and the difference between the two measurements was used to calculate the activity of SOD.
One unit of SOD was defined as the amount of enzyme that produced 50% inhibition of photochemical reduction of NBT under the assay conditions. The SOD's activity is expressed as units per milligram protein.
Catalase assay: Catalase (CAT) (EC 1.11.1.6) activity was determined as a decrease in absorbance at 240 nm for 1 min following the decomposition of H2O2. The composition of the reaction mixture (total volume: 3 ml) was as follows: 50 mM phosphate buffer (pH 7.0), 15 mM H2O2, and 20 μl of crude enzyme extract at 25 °C. The decrease in the absorbance of H2O2 was recorded at 240 nm for 2 min using a double-beam spectrophotometer, and a potassium phosphate buffer (50 mmol·L−1, pH 7.0) was used as the blank. The activity was calculated using the extinction coefficient (40 mM−1·cm−1) for H2O2 and the activity was expressed as mM of H2O2 degraded·min−1·μg−1 protein.
Ascorbate peroxidase assay: Ascorbate peroxidase (APX) (EC 1.11.1.11) activity was determined by following the decrease of ascorbate and measuring the change in absorbance at 290 nm for 1 min in 2 ml of a reaction mixture whose composition is as following: 50 mM potassium phosphate buffer (pH 7.0), 1 mM EDTA–Na2, 0.5 mM ascorbic acid, 0.1 mM H2O2 and 20 μl of crude enzyme extract at 25 °C [34]. The activity was calculated using the extinction coefficient (2.8 mM−1·cm−1) for the ascorbate [36], and was expressed as units of ascorbate oxidized·mg−1 protein.
Peroxidases assay: Peroxidase (POX) (E.C.1.11.1.7) activity was measured with a spectrophotometric assay by monitoring the H2O2-dependent oxidation of guaiacol, at 25 °C.
The reaction mixture consisted of 10 μL of 200-fold diluted 1 crude enzyme extract, 20 μL of 100 mM guaiacol, 10 μL of 100 mM H2O2 and 160 μL of 50 mM sodium acetate (pH 5.0). Control assays in which the enzyme extracts or substrates were replaced with buffer were performed. The reaction was monitored at 450 nm. One unit of POX activity (U) is defined as the amount of enzyme releasing 1 μmol of guaiacol radical·min−1 under the assay conditions.
Each result was the mean of at least three replicated treatments. Significance of differences between the treatments was statistically evaluated by STATISTICA test methods. Data were tested at significant levels of P < 0.05.
3 Results
3.1 Minimum copper inhibitory concentration (MIC) of inoculums strains
Thirty isolates were collected from nodules of Vicia faba cultivated on heavy-metal contaminated soil (Table 2). The effect of Cu on isolates growth was assessed with increasing concentrations of Cu2+. Among the isolates of the obtained collection, four strains were selected for high resistance, reaching a MIC of 2 mM Cu for SV27. In addition, strain SV23 had a maximum resistance of 1 mM Cu; however, two other strains SV15 and SV20 resistant to 0.5 mM Cu were also selected for efficiency in nodulation test.
3.2 Identification of selected isolates based on 16S rRNA gene sequence
The four bacterial isolates, selected on the basis of Vicia faba nodulation and resistance to high copper concentration, were identified by 16S rRNA gene sequence analysis. GenBank Blast searches indicated that the two isolates SV15 and SV20 were identified as belonging to Rhizobium genera. SV15 identified as R. leguminosarum bv. viciae and SV20 was identified as Rhizobium sp. CCNWSX0481. One isolate SV23 was identified as Pseudomonas sp. and SV27 as E. cloacae. Blast analysis revealed that the tested isolates were 99% similar to the Genbank match.
3.3 Bioaccumulation of copper by the selected isolates
It was established that copper accumulation differs between the cell wall and the cytoplasm after 24 h of incubation and varies between the compartments of the cell depending on the concentration of Cu (Fig. 1). The four selected strains accumulated Cu substantially when it is added in the medium at a sub-inhibitory concentration of each isolate. Indeed, the average absorption of Cu recorded in the whole cell of E. cloacae after 24 h of incubation with 0.5 mM Cu (5.44 mg·g−1 of dry biomass) was twice greater than that accumulated in the entire cell of Pseudomonas sp. up (2.44 mg·g−1 of dry biomass) (Fig. 1c and d).
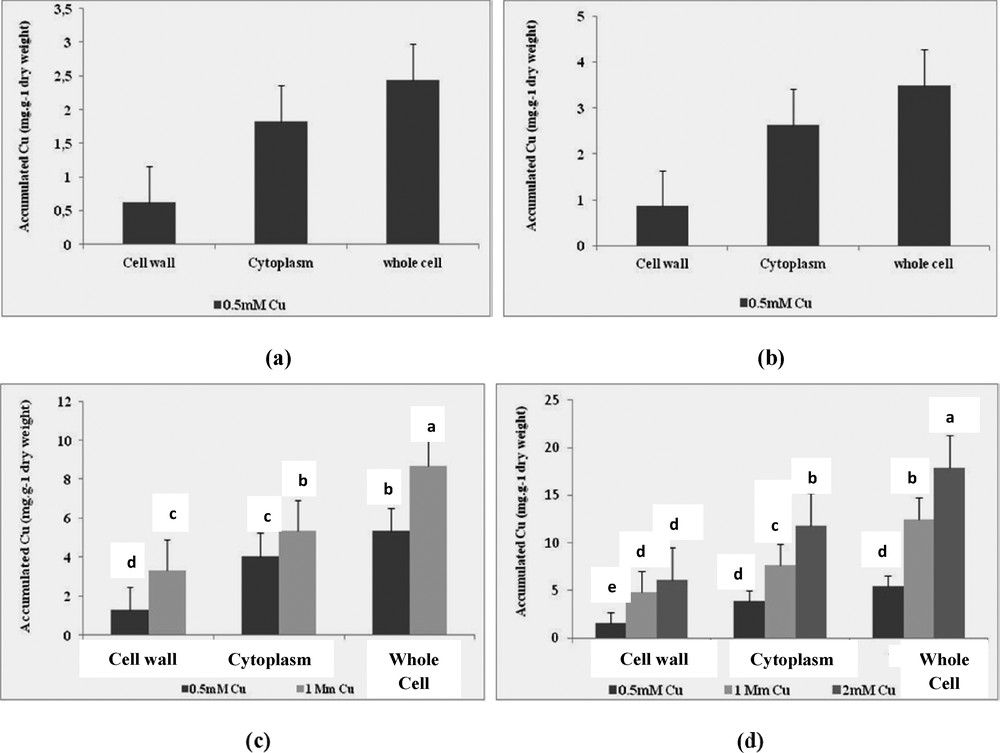
Bioaccumulation of copper by isolates (a): SV15, (b): SF11, (c): SF28 and (d): SF29 in the cell wall, in the cytoplasm and in the whole cell after 24 h of incubation in the presence of 0.5 mM, 1 mM and 2 mM Cu. Values are the means (n = 3) ± one standard error.
The highest accumulation of Cu at the cell membrane in the presence of 0.5 mM Cu (1.58 mg·g−1 dry biomass) was also noted among Pseudomonas sp.
Accumulation of copper in all the cells of Pseudomonas sp. was 8.68 mg·g−1 dry biomass after 24 h of incubation in the presence of 1 mM Cu. This value was 1.45 times lower than that accumulated by E. cloacae, which is the only featured able to grow and accumulate 2 mM Cu strain. In the case of incubation with 2 mM Cu metal accumulation in the cytoplasm, the value was 2 times higher than it was in the cell wall (Fig. 1). These results show that Cu uptake is not only due to binding to the cell's surface, but also to the intracellular accumulation and that accumulation of Cu is linked to the resistance of the metal-resistant bacteria.
3.4 Assessing the effect of co-inoculation and copper treatment on Vicia faba growth
Four days after the beginning of a 2 mM Cu treatment, symptoms of toxicity appeared in leaves of non-inoculated plants. The roots’ color changed gradually under the influence of copper ions, from creamy white to dark brown, due to the intense suberisation. Chlorosis symptoms appeared, reflecting a decrease in chlorophyll, which confirms that excess Cu is damaging to the photosynthetic apparatus.
The study of the root length in seeds co-inoculated with bacterial consortium and without Cu treatment, measured 7 days after germination, showed that the elongation was 12% higher compared to control. In contrast, root length was marked by a decrease of 17, 25 and 81% compared to their respective controls treated with 0.5, 1 and 2 mM Cu. These values were improved in the presence of inoculums (Table 3). The differences between the root length values indicated a greater resistance to copper in co-inoculated and 0.5-mM-Cu-treated plants. The addition of 0.5 mM Cu to the nutrient solution increased the shoot dry weight (SDW) by 40% and had no significant effect on root dry weight (RDW). However, co-inoculation of plants treated with 0.5 mM Cu, was without significant effect on the growth parameters, while co-inoculation improved SDW by 36% in the case of plants treated with 1 mM Cu.
Effects of inoculation and copper treatment 1 on growth criteria of 45-day-old Vicia faba plants.
Parameter | T0 | Inoculation/Copper treatment | ||||||
T0 + Inc | 0.5 mM | 0.5 mM + Inc | 1 mM | 1 mM + Inc | 2 mM | 2 mM + Inc | ||
Root length (%) of control | 100 | 112 | 82.27 | 94.16 | 74.12 | 72.67 | 18.87 | 21.28 |
Shoot dry weight per plant (g) | 2.67 ± 0.39 | 2.9 ± 0.35 | 3.76 ± 0.26 | 3.36 ± 0.51 | 2.41 ± 0.51 | 3.28 ± 0.33 | 1.03 ± 0.30 | 1.94 ± 0.38 |
Root dry weight per plant (g) | 2.87 ± 0.37 | 3.15 ± 0.44 | 3.23 ± 0.32 | 2.87 ± 0.47 | 2.63 ± 0.48 | 2.41 ± 0.20 | 1.28 ± 0.28 | 1.49 ± 0.40 |
Nodules number | 0 | 20 ± 4 | 0 | 42 ± 6 | 0 | 18 ± 7 | 0 | 10 ± 3 |
Nodules fresh weight per plant (g) | 0 | 0.014 ± 0.003 | 0 | 0.047 ± 0.009 | 0 | 0.010 ± 0.003 | 0 | 0.007 ± 0.001 |
However, 2 mM Cu treatment resulted in lower SDW and RDW by 61% and 55%, respectively. Inoculation in this case improved SDW by 88%. At harvest time, the plants treated with 0.5 mM Cu showed the highest nodulation (Table 3). This result suggests that Vicia faba has better growth with 0.5 mM Cu compared to control plants and the nodulation process has been developed for this copper concentration.
3.5 Copper uptake, coefficient of bioaccumulation and translocation factor of Vicia faba
The Cu absorption in vitro was conducted in a hydroponic medium for a period of 45 days and was estimated on a dry-weight basis. The results showed that the concentration of Cu was higher in roots than in leaves independently of inoculation. The addition of 0.5 mM Cu in the nutrient solution had no significant effect on the absorption of Cu. At 1 mM, the Cu concentration averaged 1710 and 60 μg·g−1 in the roots and leaves, respectively. In plants exposed to 2 mM Cu, the concentration of Cu is 2200 μg·g−1 in the roots and 1700 μg·g−1 in the leaves, these values were decreased by 80% and 43% after inoculation; Cu uptake was 1425 μg·g−1 in roots and 42 μg·g−1 in shoots (Fig. 2).
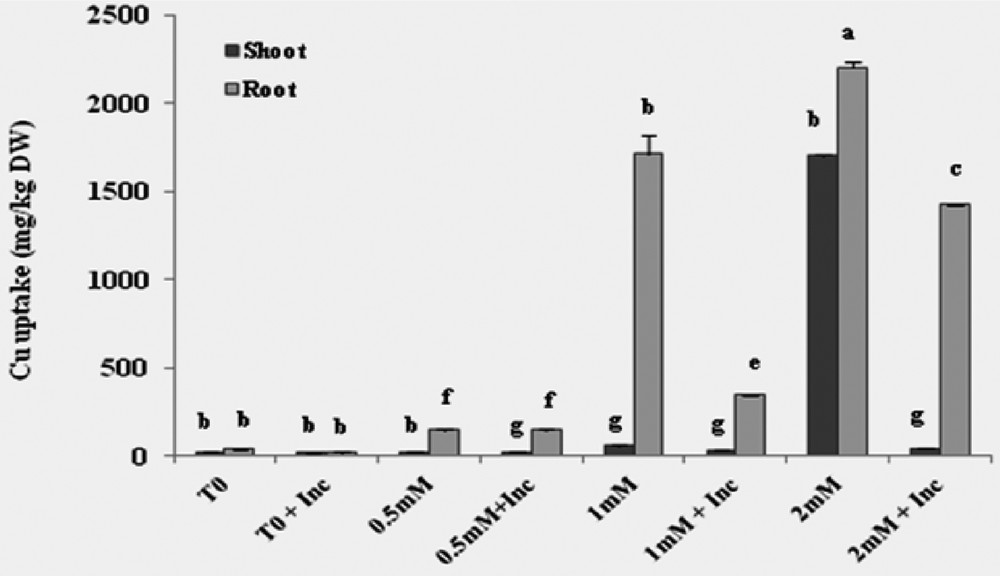
Cu accumulations in the dry biomass of roots and shoots of Vicia faba cultivated in hydroponic media with different concentrations of Cu with inoculation treatment. T0: control plants without co-inoculation and copper treatment; Inc: inoculation with the consortium of selected strains.
Cu translocation to the aerial parts is a way to maintain an acceptable concentration of copper that does not exceed a threshold value of phytotoxicity. To observe the effect of inoculation on the plants treated with copper, the coefficients of bioaccumulation and the translocation factors (TF) were studied. The results demonstrated a preferential accumulation in roots with TF values < 1, regardless of the inoculation by the consortium (Table 4). However, the values were reduced in the inoculated plants and treated with 2 mM and 0.5 mM Cu compared to uninoculated plants, particularly at 2 mM Cu. We also observed that the TF increased in plants inoculated and treated with 1 mM Cu. This result can be explained by the significant decrease in the copper concentration accumulated in plant organs. The difference in the concentration of copper transported to the aerial parts between the different copper treatments was due to the important role of co-inoculation with both PGPR and Rhizobium. However, Vicia faba tends to accumulate more copper in the roots than in the aerial parts while tolerating up to 1 mM Cu.
Bioaccumulation coefficient and translocation factor (TF) of Cu from roots to shoots of Vicia faba growing on a hydroponic nutrient solution with 0.5, 1 and 2 mM added.
[Cu] (mM) | Bioaccumulation coefficients | Translocation factor (TF) |
0.5 | 1.36 | 0.153 |
0.5 + Inc | 1.37 | 0.135 |
1 | 7.08 | 0.035 |
1 + Inc | 1.51 | 0.101 |
2 | 7.8 | 0.772 |
2 + Inc | 2.99 | 0.029 |
Furthermore, Cu depletion in the hydroponic solution at the tested concentrations of Cu has been studied. The results showed that the absorption was the highest in the first 4 days of treatment with copper and then was lowered till the 35th day of growth. The symbiosis Vicia faba inoculum absorbed up to 85% Cu from the nutrient solution after days of exposure to 0.5 mM Cu (Fig. 3). The decrease in the Cu concentration in the nutrient solution is probably due to its absorption by the resistant strains used in the inoculum.
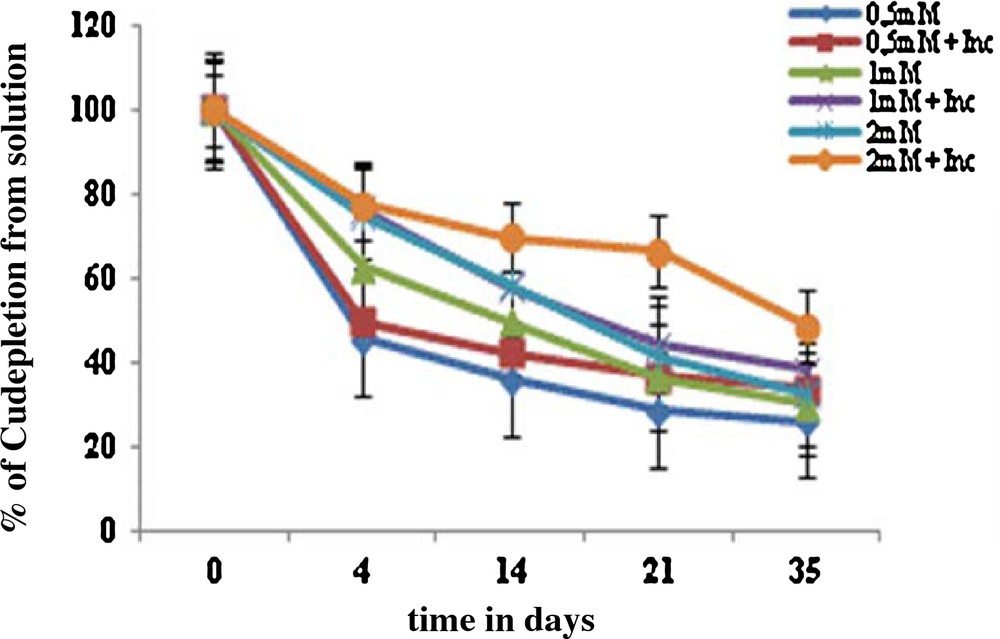
(Color online.) Depletion of copper metal from the solution shows the uptake of metal by the symbiosis (Vicia faba inoculum).
3.6 Responses of antioxidative enzymes to Cu exposure
3.6.1 Lipid peroxidation
Copper can affect the properties of the membrane by the oxidation of membrane lipids that can be estimated from the increase in MDA, one of lipid peroxidation products.
When shoot samples are concerned, the only elevation of MDA was in the 2-mM-Cu-treated plants. The inoculation in this case causes higher MDA than in shoot samples treated with 2 mM Cu alone (Fig. 4).
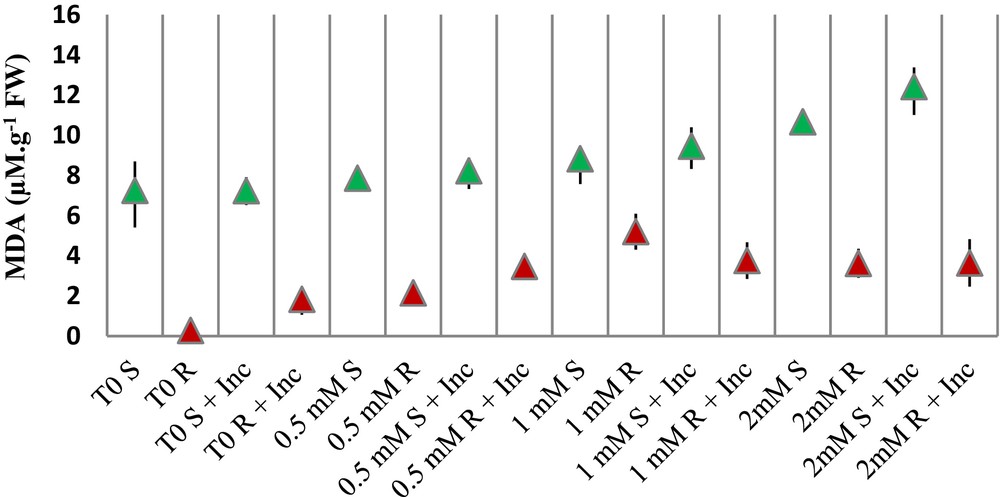
(Color online.) MDA accumulation in shoots (S) and roots (R) of hydroponically cultivated seedlings of Vicia faba treated with different concentrations of Cu (0.5, 1 and 2 mM) and inoculated with the consortium of bacteria (Inc). Data represent means and ± SD of three independent experiments.
When root samples are concerned, inoculation itself raises the levels of MDA, even without the addition of Cu (samples T0 R and T0 R + Inc). Then, the same effect is observed at the low Cu concentration of 0.5 mM Cu; it increased 7 times the concentration of MDA and inoculation induces a 61% increase. With 1 mM, there is no significant effect and with 2 mM again inoculation is related to an elevation of MDA as compared with 2 mM Cu alone (Fig. 4).
3.6.2 Antioxidant enzyme activities
There was a significant interaction between SOD, CAT, POX and APX activities in roots and leaves of Vicia faba with both cupric treatment and co-inoculation with (Rhizobium-PGPR). The different parts of the plant showed various enzymatic activities under the different concentrations of Cu (Fig. 5).
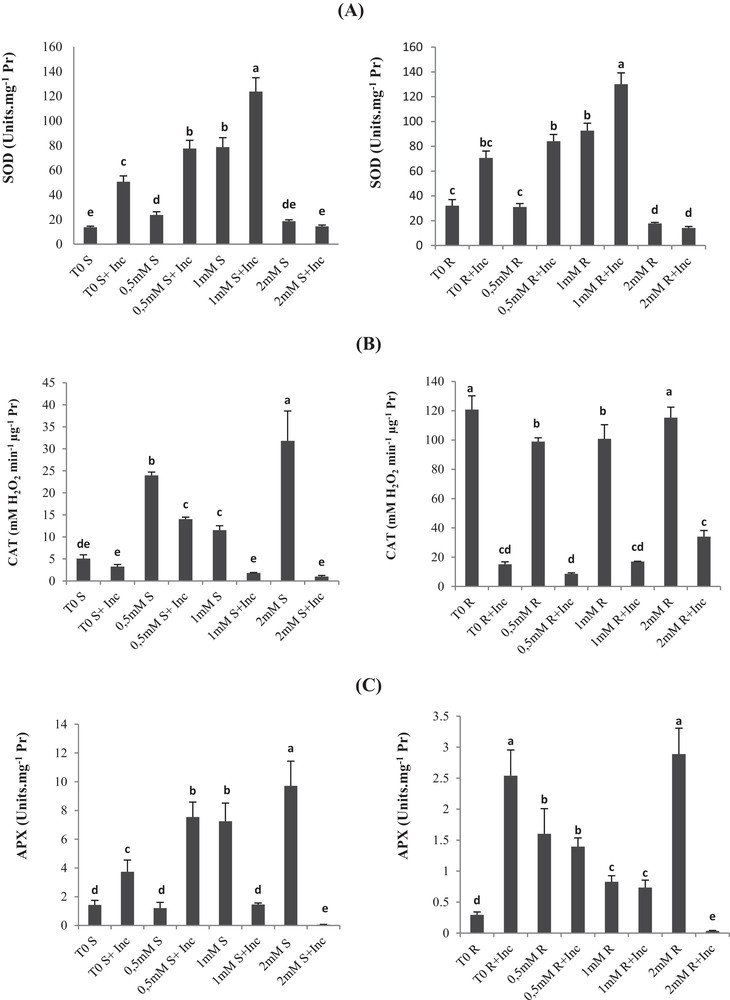
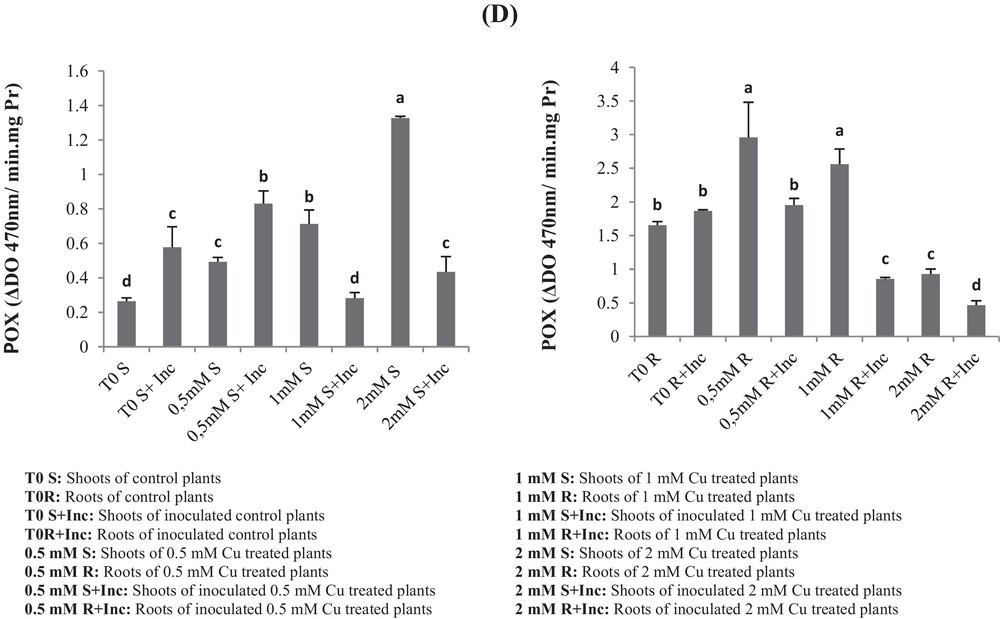
(A) Superoxide dismutase (SOD), (B) catalase (CAT), (C) ascorbate peroxidase (APX) and (D) peroxidase (POX) activities of roots and leaves in Vicia faba plants inoculated with Rhizobium and PGPR strains at different copper stress levels (mean ± SD, n = 3).
In the case of the control plants, SOD activity increased with increasing the concentration up to 1 mM Cu. The same behavior of the SOD activity was observed in the roots and leaves of 1-mM-Cu-treated and co-inoculated plants.
However, the 2 mM Cu concentration caused a decrease in SOD activity in both roots and leaves, as shown in Fig. 5a. The highest value was recorded in plants inoculated and treated with 1 mM Cu, while the lowest activity was recorded in plants treated with 2 mM Cu.
Furthermore, inoculation results in higher SOD activity for each respective Cu treatment, except for the 2-mM-Cu treatment that showed no significant difference between inoculated and non-inoculated plants.
For inoculated plants, CAT increases in 0.5-mM-Cu-treated plants as compared with the non-treated control. For non-inoculated plants, CAT increases in 0.5-mM- and 2-mM-Cu-treated as compared with the non-treated control (T0), whereas inoculation of 1-mM-Cu-treated plants showed reduced activity up to six times in 1-mM-Cu-treated plants (Fig. 5). At 2 mM Cu, CAT activity decreased significantly in the co-inoculation. Similarly, inoculation of 1-mM-Cu-treated plants inhibited until 6 times CAT activity in roots.
In shoots, the inoculation stimulated APX activities by 160% in control plants; similarly in roots, inoculation induced activation of APX by eight times (Fig. 5c). At 0.5 mM Cu, no significant effect was registered on APX activity (Fig. 5c, d). The inoculation of 0.5-mM-Cu-treated Vicia faba enhanced APX by six times.
At 1 mM Cu, APX activities in shoot samples were enhanced by five times compared to control. At this Cu level, the co-inoculation inhibited severely APX. The 2-mM-Cu APX was activated in plant shoots by a factor of 6, but inoculation inhibited significantly its activity.
In the case of roots, we registered a high elevation in APX activity by more than 5 times in plants treated with 0.5 mM Cu. At 1 mM Cu, we registered an enhancement of 181% in APX activity in roots (Fig. 5c). At those levels, the co-inoculation had no significant effect on APX activities. However, APX activity was 10-fold lower than the control in plants treated with 2 mM Cu.
In the shoots of non-co-inoculated plants, POX increased with the increasing of Cu concentration (0.5 mM; 1 mM and 2 mM Cu) as compared to the respective control T0 (Fig. 5). The activation was respectively increased by 86%, 200%, and 500% (Fig. 5d).
In the case of co-inoculated plants with the consortium of bacteria, POX increased only with the 0.5 mM Cu treatment by 68%, as compared with the respective control (T0 + Inc).
In roots we recorded an increase in the POX activity with 0.5-mM and 1-mM-Cu treatments as compared with the control T0. However, it was inhibited in plants treated with 2 mM Cu. In the case of co-inoculated roots, POX activities decreased significantly in all Cu-treated plants.
In nodules, treatment with 0.5 mM Cu stimulated SOD and POX activities by 94% and 21%, respectively. But, APX was inhibited up to 42% and no significant effect on CAT was recorded (Table 4). With 1 mM Cu, we noted the activation of SOD (46%), CAT (32%) and APX (116%), while observing a reduction of 28% in POX activity. At 2 mM Cu, all activities of antioxidant enzymes studied in nodule decreased primarily APX and POX.
These observations showed that CAT has low substrate affinity compared to POX and APX, so an alternative mode of H2O2 destruction was by peroxidases that have a much higher affinity for H2O2 than CAT in this experiment. POX activities showed an increase in response to Cu stress in root and shoot parts of both inoculated and control plants, excepted with 2 mM Cu.
The enhanced peroxidases activities in Vicia faba thus indicated an efficient detoxification of H2O2. Further, the counterbalancing of CAT and POX suggests a predominant role of POX in the antioxidative mechanism of Vicia faba. But, although POX activity increased at 2 mM Cu, it was apparently not capable of scavenging sufficient amounts of H2O2 to avoid its accumulation and related lipid peroxidation.
For all studied enzymatic activities, the maximal stimulation at 1 mM Cu treatment was observed as compared to the respective control plants. The only exception to this tendency is POX activity, where the maximum is at 0.5 mM Cu treatment. Then for all studied activities the recorded minimum is at 2 mM Cu treatment.
It can be concluded that in the nodules, SOD and POX were activated at 0.5 mM Cu; however, treatment with 1 mM Cu improved SOD, CAT and APX activities. In consequence, there was no antioxidant enzymes activation in roots of 0.5- and 1-mM-Cu-treated plants.
Moreover, in aerial parts, Vicia faba responded to copper stress by SOD, APX and POX enhancement at 0.5 mM Cu and by activation of only SOD and APX at 1 mM Cu. Treatment with 2 mM Cu caused severe plant damages and inactivated all the antioxidant enzyme system of the legume (Fig. 6).
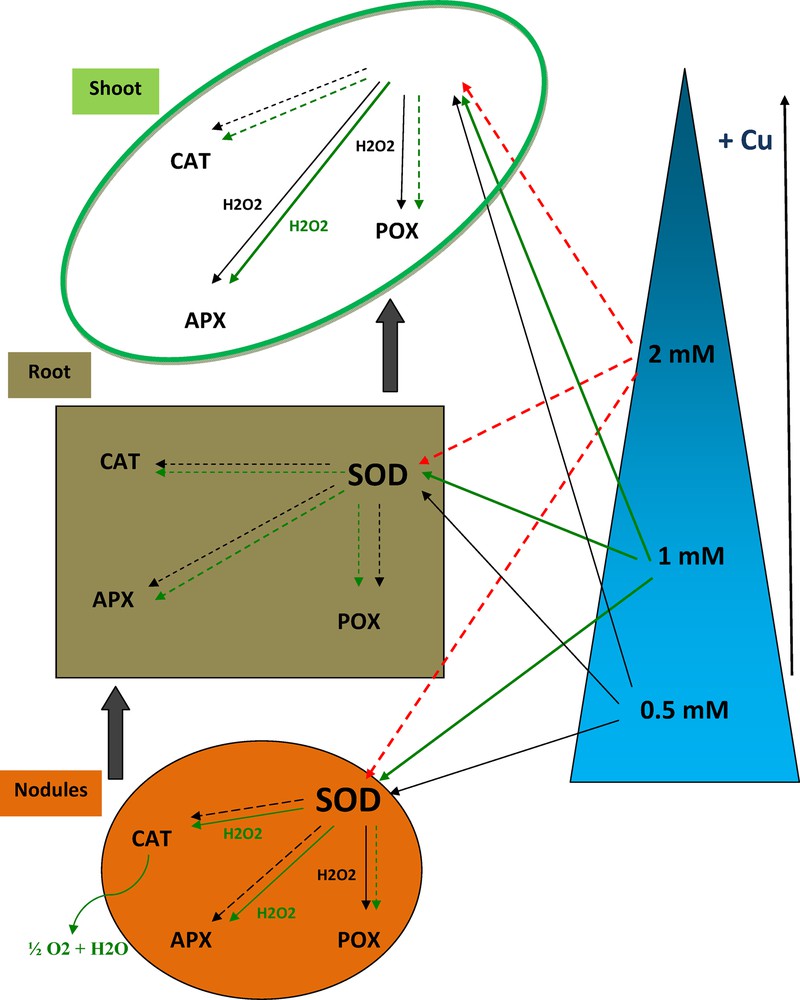
(Color online.) Effects of different Cu concentrations on SOD, CAT, APX and POX activities in co-inoculated Vicia faba shoots, roots and nodules.
4 Discussion
4.1 Copper tolerance and accumulation by selected strains
Recently, the application of PGPR has been extended to the bioremediation of metal pollutants, and Rhizobium legume has an extra advantage due to nitrogen fixation in plant root nodules [37]. In the present study, co-inoculation of Vicia faba legume was conducted with four strains Rhizobium sp. CCNWSX0481, R. leguminosarum bv. viciae, E. clocae and Pseudomonas sp., characterized by efficiency and copper tolerance [38]. The selected bacteria have different levels of resistance to copper with the highest MIC observed in strains of Pseudomonas sp. and E. cloacae. Our results are consistent with those showing that Enterobacter sp. P36 is able to tolerate high Cu (2 mM) and have multiple PGPR traits as promoting plants growth in copper-stress conditions [39]. This finding is comparable to other works showing copper-resistant bacteria belonging to Acinetobacter, Pseudomonas, Enterobacter, Ensifer meliloti [40,41].
In our study, two of the selected isolates were not only resistant against copper but also had the capability of accumulating this metal. It is well reported that microorganisms have an affinity for metals and can accumulate them by various mechanisms [42].
The rates of bacterial growth and copper accumulation were related to copper concentration. Increasing copper concentration lowered growth due to its toxic effect; however, increasing concentration increased copper removal. A similar relationship between copper uptake and concentration was observed by [43] and alike correlation between concentration and bioremoval was reported for the Pseudomonas sp. strain NA having the capacity to reduce and remove copper at the highest concentration of 25 mg·l−1 [44]. In the present study, two copper-resistant strains were obtained from the legume Vicia faba grown on contaminated soil and were identified as Pseudomonas sp. and E. cloacae. The interesting features of those strains include the presence of copper in bacterial cytoplasm showing accumulation ability. The uptake of copper in the whole cells of strain E. cloacae of 18 mg·l−1 in 24 h was much higher than it was by the isolate A. tumefaciens CCNWRS33-2 that removed 6.35 mg·l−1 of copper after 36 h [45]. However, it was lower than bioremoval of Staphylococcus pasteuri N2 isolated from vineyard soil that accumulated 80 mg·l−1 after 24 h [46].
Co-inoculation with selected strains may also help reducing the toxicity of copper to Vicia faba in polluted environments. In fact, recent results showed PGPR ability for improving soil structure and bioremediating the contaminated soils by sequestering toxic heavy metal species [47].
4.2 Inoculation effect on Vicia faba under cupric stress
Many different vegetative response endpoints such as morphological behavior, root length, shoot height and total biomass are used to indicate metal resistance to plants [43]. Our study showed that a concentration of 0.5 mM Cu had a benefic effect on Vicia faba growth and nodulation. However, treatment of 2 mM Cu was found to be toxic, inhibiting root elongation and ramification. This result is an agreement with those showing that copper toxicity results in reduced root length, root hair inhibition and reduction in fresh weight [44]. Moreover, the root system was found to be more sensitive to copper toxicity due to the direct effect on root [45]. Marques et al. [46] described also the effect of high copper levels on the legumes leading to the induction of leaf chlorosis, the reduction of root growth, and the inhibition of both elongation and division cells.
Metal toxicity to plants can be alleviated by introducing metal tolerant plant-growth-promoting bacteria (PGPB) that are able to promote plant growth under stress conditions [47]. The present results indicated that inoculation of 0.5-mM-Cu-treated plants had no significant effect on the studied growth parameters, whereas the inoculation enhanced the SDW of those treated by 1 mM and 2 mM Cu. In other findings, co-inoculation of lupines with a consortium of metal-resistant PGPR produced an additional improvement of plant biomass [48]. Moreover, the inoculation of Medicago lupina with Sinorhizobium meliloti CCNWSX0020 significantly increased plant biomass and copper concentration inside the plant tissues [49].
4.3 Copper uptake, coefficient of bioaccumulation and translocation factor of Vicia faba
The present results indicated that copper accumulated predominantly in roots, as compared to the shoots of the studied Vicia plants, suggesting that metal accumulates immediately in roots, probably later on translocated to shoots and leaves. The present work showed also a correlation between absorbed copper and copper level in the medium, so we suggest that the metal was sequestered primarily in the root system. These results are consistent with those obtained by [34]. Recently, PGPB are shown to alleviate metal toxicity to plants by reducing accumulation in plant parts [50,51]. In the present work, the translocation factors were significantly reduced in the inoculated plants, especially those treated with 2 mM Cu.
These results suggested that the inoculum acts in Vicia faba plants as a copper filter to maintain low concentrations in the above ground plant tissues. In this connection, Wani et al. [52] reported that strain Proteous vulgaris reduces copper accumulation in pigeon pea. The reduction of Cu concentration in the nutrient solution is probably attributed to copper-tolerant strains of the inoculums. In this context, it has been reported that effective phytoremediation could be accomplished by bacteria having the potential of solubilizing heavy metals [53].
4.4 Lipid peroxidation and responses of antioxidative enzymes to Cu exposure
Our results showed that excess Cu increases oxidative stress which is manifested by increased lipid peroxidation. This finding is in agreement with the preceding results showing that MDA has accumulated substantially after exposure to Cu and the cell membrane is the principal site of Cu toxicity [54]. Other authors have shown an increase in tissue MDA in response to Cr [55], Pb [56], and Cd [57]. In agreement with our data, this could be due to the overproduction of ROS stress Cu, which is highly destructive to the cell membrane. In this work, the results suggested that Vicia faba, in which an effective antioxidant enzyme is involved, has the ability to tolerate the stress up to 1 mM Cu. Similar results have been reported in Arabdopsis thaliana exposed to copper stress [58]. The response of CAT enzyme in Vicia faba showed a more pronounced increase in nodules than in roots and shoots of inoculated and Cu-treated plants.
However, the lack of variation in activity in roots suggesting that CAT appears not to be required for the elimination of H2O2, as it has been reported in rice plants [59]. These conflicting observations regarding CAT activity may be due to differences in the plant organs studied, plant growth conditions, and the exposure time to different concentrations of the metal used [60]. The inhibition of CAT activity under stress may also be ascribed to more consumption of enzyme to detoxify H2O2 or its inactivation [61]. Antioxidant enzymes activities declined significantly in inoculated plants when treated with 1 mM Cu. In this case, nodules play an important role by the activation of SOD, CAT, and APX. Elsewhere, the nodules served as metal buffer zones, providing extra protection for the plant against invading metal ions and other soluble contaminants, since the heavy metal would inevitably make contact with the nodules [62].
At 2 mM Cu treatment, the metal had toxic effects on Vicia faba plant, which is in agreement with research observing that copper excess damages cells by causing oxidative damage through the generation of reactive oxygen species [63]. APX and POX involved in the detoxification of H2O2 increased with increasing the Cu stress, but their activities decrease at 2 mM Cu. A similar APX decrease was observed in Cd-treated pea [64]. This toxicity resulting from the cellular oxidative stress may be allayed by several antioxidant systems. However, the activities of the antioxidant enzymes were affected differently.
The activity of antioxidant enzymes in Vicia faba roots and shoots decreased significantly under copper stress after co-inoculation, suggesting that this inoculum could be used for the amelioration of Cu stress in Vicia faba legumes. The obtained results are consistent with others indicating that the co-inoculation with B. subtilis and Arthrobacter sp. could alleviate the adverse effects of soil salinity on wheat growth [65].
5 Conclusions
Biological inoculums for legumes have attracted much attention throughout the world. PGPR inoculants are available for a variety of crops, used alone or co-inoculating with Rhizobium sp. The findings of the present study suggest that the used inoculum can not only improve plant biomass, but also resist to the toxic effects of Cu contamination. This beneficial effect could be attributed to the decrease in the Cu level inside the roots and shoots of Vicia faba plants in symbiosis with tolerant and accumulating Cu strains. It can be concluded also that low Cu treatment interfere significantly with the growth and development of Vicia faba, before reaching toxic levels. It acts as a promoter of growth as it is an essential element, but induces oxidative stress with increasing concentration (> 2 mM).
Although the activity of antioxidant enzymes was significantly increased by copper stress, the inoculated plants showed the best growth performances and reduction in enzyme activities. This inoculum can be suggestible for plants in moderately copper-contaminated conditions. This technology has resulted in positive responses under controlled conditions; however, natural variations make it difficult to predict how the inoculums may respond when applied to field conditions. Strains must be propagated artificially to optimize their viability and biological activity under field applications.
Disclosure of interest
The authors declare that they have no conflicts of interest concerning this article.
Acknowledgements
The authors thank Pr. Moez Jebara director of legumes laboratory for allowing them to carrying out this research. Omar Saadani (O.S.) carried out most of the experiments linked to antioxidant enzyme activities: preparation of plant samples and spectrophotometer essays. Manel Chiboub (M.C.) coordinated some experiments (screening of bacteria, analysis of heavy metal tolerance and molecular characterization). Salwa Harzalli Jebara (S.H.J.) made substantial contributions to the conception, the analysis and the interpretation of the results. All authors read, approved the final manuscript and took part in the discussion about this work.