1 Introduction
Trees regulate the spatial position of their axes in response to environmental cues, by means of active dynamic longitudinal growth stresses [1]. These stresses responsible for stem reorientation, result from an asymmetric radial growth due to an increase in the number of division of the cambial initials, coupled with the differentiation of a particular wood made of specialised xylem cells and referred to as reaction wood. Reaction wood characteristics and location differ between angiosperm and gymnosperm trees. In gymnosperm trees, such as pine, the reaction wood is named compression wood and develops at the lower side of leaning stems and branches, whereas in angiosperm trees, such as poplar, it is named tension wood and occurs at the upper side. Both in angiosperms and gymnosperms, there is a similar differential lignification of leaning stems, with a greater lignification on the lower side [2]. Hence, lignification, among other features, is thought to be important in reaction wood both for its formation and the definition of its properties. Whereas the importance of lignin in the definition of compression wood mechanical properties is well established [3], this is far from being the case for tension wood. In the first part of this review, we report on the distinctive features of the cell walls in tension wood, with particular reference to lignin and lignification. In the second part, we discuss the potential role of lignin in tension wood properties and propose several directions to investigate in order to improve our knowledge on this topic.
2 Characteristic features of tension wood cell walls
2.1 Tension wood anatomy
This is mainly the wall of reaction wood fibres that exhibits specific biochemical, ultra-structural, and mechanical features. In the primary wall of xylem cells, cellulose microfibrils are randomly oriented, whereas, in the secondary wall, they are highly oriented, being fixed by the hemicellulose gels to form a rigid, twisted honeycomb structure [4]. Lignification occurs firstly at the cell corner and middle lamella, then extends to the secondary wall. Therefore, the cell wall can be assimilated to a composite made of a matrix of lignins and hemicelluloses, reinforced by long bundles of cellulose microfibrils [5]. Tension wood anatomy is derived from this general pattern and varies depending on hardwood species. Using image analysis technology, Jourez et al. [6] gathered a large number of data on the anatomy of tension wood and opposite wood on poplar cuttings induced to form tension wood upon a gravitational stimulus. Both fibres and vessels were significantly longer in tension wood, which may result from a prolonged differentiation of tension wood cells in comparison to normal xylem cells. These authors also observed a significant reduction in the number and size of vessels and a corresponding increase in the proportion of fibres that exhibit very thick cell walls. Indeed, for a lot of hardwood species, the major anatomical characteristic of tension wood is the occurrence of xylem fibres with a thick extra layer at the inner side of the secondary wall, which is not very cohesive with the other cell wall layers (Fig. 1) [2]. Because of its translucent and gelatinous appearance, this extra-layer has been named G-layer. G-fibres (tension wood fibres containing a G-layer) have on average a smaller radial diameter than normal fibres in tension wood [6]. It must be pointed out that this G-layer is absent from the tension wood of a number of hardwood species: for example, among 346 Japanese hardwood species surveyed, G-layers was not found in 137 (about 40%) of them [7]. However, in species without G-layer, the S2 layer tends to develop features related to those of the G-layer that are described thereafter [8].
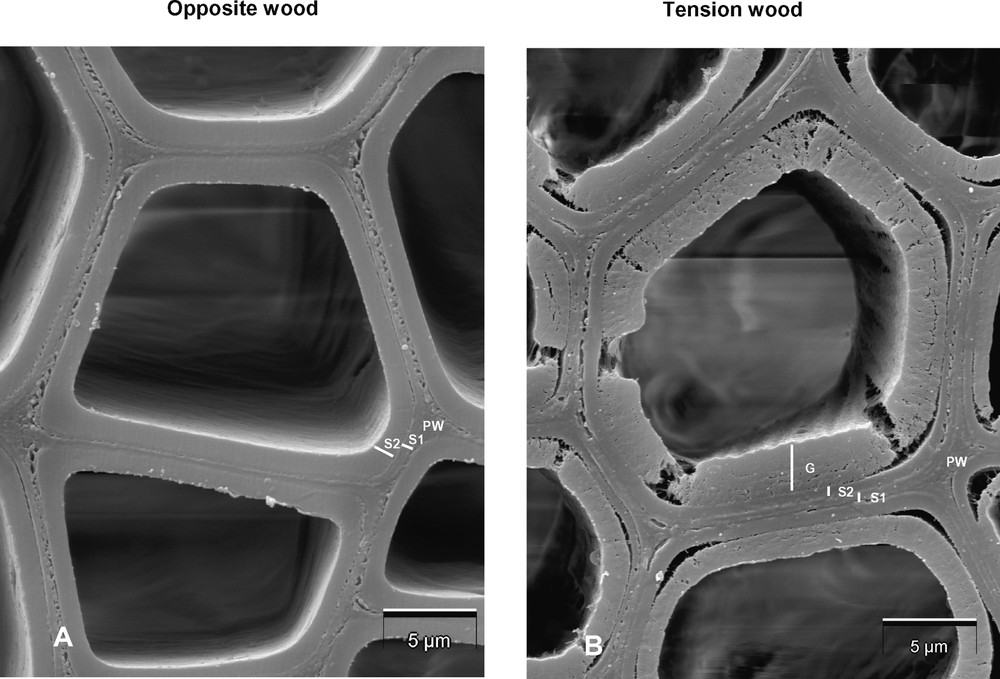
Scanning electron microscopy in fibres of poplar wood: (A) in a wood area opposite to tension wood; (B) in tension wood. Note the inner thick gelatinous layer (G) not very cohesive with the two other secondary wall layers S1 and S2, which appear thinner than in opposite wood. PW: primary wall and middle lamella.
2.2 Tension wood at the biochemical level: is there less lignin in tension wood?
Tension wood is generally described as containing a high proportion of cellulose and being less lignified than normal wood [9–11]. In fact, whereas the vessel cell wall appeared generally well lignified, this is mostly the fibre cell wall that seems partly unlignified.
From analyses performed on ten hardwood species, Timell [12] determined an average chemical composition of tension wood and concluded that the lower overall lignin content generally measured in tension wood results from the incorporation of more cellulose in the G-fibres. Indeed, when expressed on the basis of equal cellulose content, tension wood, in comparison with normal wood contained less glucomannan, two to five times as much galactan [13,14], an equal or a slightly lesser amount of xylan, and the same quantity of lignin. It appears that the parts of the cell wall in tension wood that are deposited prior to the development of the G-layer, usually the S1 and S2 layers contain at least as much lignin and xylan as in normal wood. Moreover, as S1 and S2 layers are generally thinner in tension wood fibres than in normal fibres, Timell [12] indicated that the concentration of lignin must be considerably higher in these layers than in the corresponding layers of a normal fibre. Timell concluded that a lack of lignification should not be regarded as characteristic of tension wood as a whole but only of the G-layer.
Indeed, the G-layer is described as almost entirely composed of cellulose, with highly crystalline microfibrils oriented nearly parallel to the longitudinal axis [2]. Histological staining reactions, such as safranin-light green or phloroglucinol–HCl, seem to indicate that the G-layer is usually non-lignified or lignified to a very limited degree, whereas the wall layers external to the G-layer are strongly lignified.
Norberg and Meier [15] succeeded in the isolation and characterisation of pure G-layers by means of an ultrasonic treatment. The qualitative sugar analysis of a hydrolysate of G-layers strongly indicates a purely cellulosic nature of the G-layer, but these authors did not perform any phenolic analysis. Using UV microscopy and staining techniques, Scurfield and Wardrop [16] observed in several species that the G-layer could sometimes be partly lignified, especially in transitional regions between normal and tension wood. This has been also reported by Araki et al. [17] in Robinia pseudoacacia and Populus euramericana using transmission electron microscopy and KMnO4 staining. Using UV microspectrophotometry, Yoshida et al. [18] detected lignin in small amount in the G-layers of tension wood fibres of black locust. Lignin has also been immunocytochemically detected in the G-layer of poplar tension wood [19]. Therefore, in tension wood, lignification seems to be reduced only in the G-layer.
2.3 Is there modified lignin in tension wood?
Early studies performed on different hardwood species have consistently identified a correlation between Klason lignin content and lignin composition: high guaiacyl lignin correlate with high lignin contents and conversely low lignin contents correspond to high syringyl lignin. This relation seems to apply in tension wood: in 1971, Sarkanen and Hergert [20] already reported that tension wood in birch and madronna exhibits both a lower lignin content and more S units than normal wood. Likewise, Bland and Scurfield [21] using autoradiography demonstrated a lesser extent of methylation in opposite wood compared to tension wood.
Although a few reports (e.g., [22]) are contradictory, most of the studies agree on an increased S/G ratio in tension wood. Yoshida et al. [23] determined in situ lignin content using UV microspectrophotometry in yellow poplar, a tree species that does not form G-fibres, but whose secondary wall becomes gelatinous layer-like in the area with high tensile strength. They correlated an increased tensile strength, indicative of tension wood area, with a decreased microfibril angle (or MFA, that is the angle of cellulose microfibrils with the fibre axis) and both a decreased lignin content and an increased S/G ratio. Similar results were obtained in black locust [18], poplar [11], and eucalypts [24,25].
Recent reports indicate that the low amount of lignin present in the G-layer is also enriched in S units. Using antibodies specifically directed against non-condensed lignin, Joseleau et al. observed by immunogold electron microscopy the presence of guaiacyl-syringyl lignin epitopes within the G-layer of tension wood fibres [26]. Moreover, using another antibody specific for S units (Fig. 2), they estimated that the abundance of S units into the G-layer was about 40% of that in S2. Using microspectrophotometry coupled with the Maüle reaction (Yoshinaga, unpublished results) was also able to detect such a small amount of lignin rich in S units in the G-layer of poplar tension wood fibres.
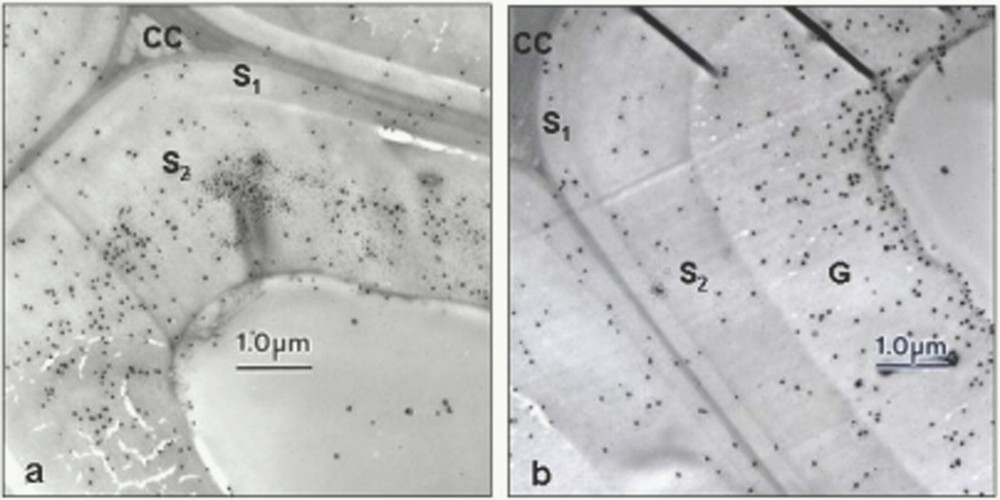
Transmission electron microscopy immunolabelling of S epitopes of lignin in fibres of poplar wood: (a) ultrathin cross-section of a normal xylem fibre (syringyl epitopes appear distributed in S1 and S2 with a higher concentration in the inner part of S2); (b) G-fibre (syringyl epitopes are present in S1, S2 and G layers and particularly concentrated in the inner part of the G-layer).
It has been proposed that the increased S/G ratio generally observed in tension wood results from an enzymatic regulation. Scurfield [27] already reported an increase in peroxidase activity in the G-layer of living reaction wood cells. Aoyama et al. [25] observed an increased activity of wall-bound peroxidases that catalyse preferentially syringyl polymerisation during tension wood formation in Eucalyptus viminalis (a species that differentiates G-layers). A similar preference of cell-wall-bound peroxidases for S unit has also been observed in poplar tension wood [28].
Finally, Akiyama et al. [29] reported a slight but significant increased proportion of the erythro form in β-O-4 structures (the predominant linkage types in lignin) in the tension wood of yellow poplar, which strongly correlated with an increased content in methoxyl group of the lignin.
All these data suggest that lignification in G-layer is specifically regulated in favour of syringyl units. It remains to determine what could be the effects on the cell wall mechanical properties of the syringyl enrichment in G-layer lignin and if this effect results from a higher proportion of the erythreo form of β-O-4 structures in tension wood lignin.
2.4 May lignin characteristics in tension wood be influenced by polysaccharides?
Although the lignin polymer is often considered as a random network, its deposition seems to be spatially and temporally regulated [5]. Among other factors, it has been proposed that both the chemical nature of the carbohydrate matrix and the orientation of cellulose microfibrils may influence lignin deposition [30].
During the assembly of the secondary cell wall, the polysaccharide matrix is always deposited prior to lignification: this suggests a coordinated regulation of lignin and cellulose deposition. Siegel [31] already pointed out the importance of the nature of the polysaccharide matrix in which lignin is polymerised: he proposed that the association of lignin precursors with the polysaccharide surfaces might result in some organisation of these precursors prior to polymerisation. Houtman and Atalla [32] suggested that lignin, although hydrophobic, could adsorb on polysaccharide surface such as cellulose microfibrils. They proposed that polysaccharides, both cellulose and hemicelluloses, are templates for the assembly of lignin and therefore polysaccharide composition would control lignin structure. In their view, different sugar moieties will interact with lignin precursors in different ways or to different degrees. Such interactions may provide the basis for selective binding of the different lignin precursors in a manner that can impart spatial organisation to the lignin.
In their model of lignification in restricted spaces, Roussel and Lim [33] found that the geometry of the hemicellulose matrix is an important factor. Besombes and Mazeau (unpublished results) investigated the interactions between cellulose and lignin at the atomistic scale using molecular modelling. They identified hydrophobic interactions between lignin aromatic rings and apolar CH groups of cellulose. Importantly, these interactions lead to a preferential parallel orientation of lignin aromatic groups relative to cellulose surface, which appeared linked to the molecular mass and the structure of lignin. Notably, these authors found that the presence of branching and some particular inter-unit linkages were a hindrance to this parallel orientation.
From these general studies on lignin–polysaccharide interactions, it is tempting to speculate on potential links between the very peculiar polysaccharide composition of the G-layer highly crystalline cellulose, low MFA, increased galactan and decreased xylan, and the lignin modifications generally observed in this layer.
3 A role for lignin in tension wood properties
Despite all the studies performed to understand the mechanisms involved in the development of tension wood, what Timell wrote some 30 years ago still applies today [12]. We still do not know precisely how exactly reorientation occurs. For a long time, it has been postulated that the stem of a tree may be regarded as a viscoelastic body capable of deformation over long periods of time and that the formation of tension wood was associated with the development of important longitudinal growth stresses. After some controversy, it is now generally admitted that (i) such stresses result from modifications in the structure and chemical composition of the cell wall during tension wood formation and (ii) the bending of hardwood stems and their subsequent reorientation directly result from these stresses. However, the relations between tension wood characteristics and the stress generation remain unclear and, among these characteristics, the role of lignification is still a matter of debate. As the cell walls have the capacity to be stressed and to exert forces only after they have acquired stiffness and elasticity, the stresses responsible for stem orientation should develop while the young xylem cell walls are solidifying upon lignification [34].
A first explanation for the development of longitudinal growth stresses is based on the observations that (i) during differentiation, fibres and vessels have a tendency to contract in length and to expand laterally and (ii) the degree of this contraction is less when the lignin content in the cell wall is high.
In the lignin swelling hypothesis [35], Boyd explains the simultaneous generation of longitudinal tension and tangential compression by one single process: the deposition of encrusting lignin between cellulose fibrils that causes transverse expansion of tension wood fibres. Because of the spiral arrangement of the microfibrils, this transverse expansion is associated with longitudinal contraction, just as a wetted rope of twisted natural fibres shortens [34]. Since this contraction is restrained by older wood, the cells newly formed generate longitudinal tension, while obstruction of the lateral expansion by neighbour cells leads to tangential compressive stress [36]. From these observations, it has been assumed that forces would be generated from fibre contraction that will lead the stem to become concave toward the less lignified side. The specific ultrastructure of tension wood would enhance this bending induced by a differential lignification. Since, in tension wood, lignin is largely located in the layers external to the G-layer, it should be these layers that swell upon lignification. The force so created is mainly directed transversely by virtue of the resistance offered by the G-layer to longitudinal expansion. This hypothesis is consistent with the evidence of lateral compression of vessels and parenchyma cells generally observed in tension wood area. This is also in accordance with the poor lateral cohesion of the G-layer with the outer wall layers of the fibres. In front of this hypothesis, the main objection was on the reality of the swelling of the secondary cell wall. In 1978, Bamber [37] proposed an alternate hypothesis, the cellulose tension hypothesis.
As already suggested by Wardrop [2], tension arises from the contraction of crystallizing cellulose during secondary wall formation. Both the formation of the microfibrils and their simultaneous polymerisation would be responsible for the fibrils to contract longitudinally. Lignification, as an inhibitor of cellulose crystallisation, would reduce the longitudinal contractions.
The growth stress generation is also affected by the diurnal change of turgor pressure during cell wall maturation [37]. This variation in turgor pressure induces an irreversible elongation of the cells. The newly produced cell wall will have its cellulose microfibrils stretched or loosened by turgor pressure changes. As a consequence, lignin precursors will penetrate more or less easily for deposition in the gaps between microfibrils. Therefore, the stretched cellulose microfibrils under high turgor pressure cannot return entirely to their original state as a consequence of obstruction by adhesive force of adjoining cells and also by lignin and hemicellulose deposition between microfibrils. The repetition of this process accumulates residual tensile stress in the axial direction of microfibrils and compressive stress in their lateral direction.
The cellulose tension hypothesis fits well with the small lignin and large cellulose contents present in the G-layers.
The controversy between Boyd and Bamber's hypotheses remained unsettled [38]. Recently, however Okuyama et al. [4] proposed a ‘unified hypothesis’, where growth stresses result from the interrelations between the tensile stress of cellulose microfibrils generated positively in their axial direction and the compressive stress that is generated by the deposition of lignin into the gaps of the microfibrils.
Models derived from the mechanic of composite materials emphasise the importance of MFA, crystalline cellulose content and the mechanical properties of the matrix to explain at the macroscopical level the variations in the mechanical properties of wood. According to these models, longitudinal tensile stress decreases when the MFA or the lignin content increases, and increases with the crystalline cellulose content.
In support of either of these hypotheses, a number of authors have experimentally established relations between tension wood mechanical properties and the ultrastructure and chemical composition of the cell wall. For example, in a study on seven hardwood species, Okuyama et al. [4] correlated extremely large longitudinal tensile stresses with the occurrence of gelatinous layer, in species that differentiated such layers. In species without G-fibres, they found a positive correlation between tensile growth stress and MFA, but failed to establish a strong correlation between stress and lignin content. These results were confirmed in recent analyses performed on other species with or without G-fibres [18,23]. In another work, performed on a eucalyptus species that do not differentiate G-fibres, Baillères et al. [24] established good correlations between longitudinal growth stress and both MFA and lignin content (
It is worth noting that the different proposed model and theories merely do not take into account the very peculiar structure of G-fibres. If in the G-fibres, the ‘cellulose tension hypothesis’ best apply to the G-layer, whereas the ‘lignin swelling hypothesis’ seems more relevant to the surrounding S1 and S2 layers, there is a general lack of knowledge on any potential interactive effects between layers. We are in a need for models that will consider the particular arrangement of the G-layer with high crystalline cellulose and low MFA, entangled in the S1 and S2 layers with higher or normal lignin concentrations, and predict the effects of this structure on the mechanical properties of tension wood. Such a model would certainly help to elucidate what advantage, if any, does the development of a G-layer bring about, and why some species differentiate such a G-layer when others just modify their S2 layer.
4 New studies based on functional genomics
The differentiation of tension wood is certainly due, at least in part, to variations in the expression patterns of a set of genes. For several years, genomic studies have brought over new knowledge on the genes involved in wood formation.
4.1 Sequencing projects
Thanks to the development of high-throughput sequencing technologies, it has been possible to generate a high number of expressed sequence tags (EST), reflecting the population of genes expressed in different types of wood, which lead to the discovery of genes specifically involved in wood formation. With the recent sequencing of its genome (http://genome.jgi-psf.org/poplar0/poplar0.home.html), poplar is now the model species for genomic studies focused on tree specific features such as wood formation. Today, more than 140 000 EST from Populus have been sequenced from a wide variety of tissues and are available in public databases [40,41]. Sterky et al. [42] were the first to report the sequencing of 5692 non-redundant EST from wood-forming tissues in poplar species. In a similar study focused on tension wood formation, Déjardin et al. [43] have sequenced more than 10 000 EST corresponding to genes expressed in woody tissues from trees induced to differentiate tension wood. The analyses of these EST gave a picture of the genes expressed in the differentiating xylem both in tension and opposite wood. In tension wood, different genes coding for arabinogalactan proteins (AGP) were strongly over-represented as well as genes from cellulose biosynthesis. AGP are highly glycosylated proteins whose function remains unclear. They are strong candidates to be mediators of cell–cell interactions and regulators of cell growth [44]. Recently, an Arabidopsis AGP mutant has been shown to exhibit perturbed cell walls and an increased sensitivity to salt stress that causes the swelling of the cells in root elongation zone [45].
Among lignin biosynthetic genes, a CCoAOMT gene was significantly under-represented in the tension wood, suggesting a lower level of expression in tension wood xylem. Interestingly, Chen et al. [46] have demonstrated in poplar that two CCoAOMT promoters (corresponding to the two CCoAOMT genes identified in poplar) were responsive to mechanical stress. Indeed, upon bending or leaning of the stem, their expression was induced in all cell types whereas without stress, their expression seems to be restricted to vessels and contact rays. Unfortunately, this study has been performed only nine days after the application of the mechanical stress and the formation of tension wood was not yet anatomically visible [46].
4.2 Proteomics
Only a few studies have been carried out at the protein level on tension wood formation. Baba et al. [47] identified five different proteins attached to the cell wall and the plasma membrane that were specifically present in tension wood tissues, two weeks after induction. Likewise, Plomion et al. [48] identified in E. gunii 12 different proteins significantly associated with growth strains. All these proteins still need to be identified by microsequencing or mass spectrophotometry.
4.3 Transcriptomics
As exemplified by Hertzberg et al. [49], DNA microarray allows for determining the pattern of expression of a high number of genes during wood formation. Preliminary experiments on global gene expression in tension wood performed in INRA–Orléans (Leplé et al., unpublished results), confirmed the over-expression of several AGP genes and of genes coding for enzymes from the cellulose synthase complex already detected after EST sequencing [43]. Global gene expression has also revealed that a number of other genes are regulated during tension wood formation such as, as already shown [50], a gene coding for amino-cyclo-propane (ACC) oxidase that is strongly over-expressed in tension wood. Important differences between the expression of lignin biosynthetic genes were also recorded (Table 1). Two phenylalanine ammonia-lyase (PAL), a 4-coumarate-CoA ligase (4CL), a caffeic acid O-methyltransferase (COMT), a caffeoyl-CoA O-methyltransferase (CCoAOMT), a ferulate-5-hydroxylase, a cinnamyl alcohol dehydrogenase (CAD) and a peroxidase appeared highly expressed in wood, whereas cinnamoyl-CoA reductase (CCR), two trans-cinnamate 4-monooxygenase (C4H) and a laccase exhibited only low levels of expression. More interestingly, among the highly expressed genes, only the two PAL genes and a 4CL gene were strongly (2 to 4 times) down regulated in tension wood. The expression of the genes coding for the enzymes involved in the synthesis of syringyl units (F5H, COMT and CAD) does not appear significantly affected in tension wood. Due to their low level of expression, it was not possible to conclude on the pattern of expression for CCR, C4H and laccase genes. Although, these results still need to be confirmed, they suggest that both the reduced lignin content and the increased in syringyl units observed in the G-layer may result from a change in the expression of genes coding for enzymes of the lignin biosynthetic pathway. Indeed, transgenic plants down regulated for either PAL or 4CL (both strongly down-regulated in tension wood) have been shown to exhibit lower lignin content with a preferred reduced content in G units [51,52]. PAL and 4CL genes have also been shown to be up regulated in Pinus taeda cell cultures upon phenylalanine addition [53]. This suggests that lignin reduction in tension wood may result from a shortage in lignin precursors due to a decrease in phenylalanine availability.
Summary on the level of expression of genes coding for enzymes from the lignin metabolism in tension or opposite wood, 28 days after gravitational induction. For a same enzyme, different Genbank accessions correspond to different transcripts. The values are the average of three measurements per slide in arbitrary unit after normalisation to remove background levels (Leplé et al., unpublished results)
Genebank Acc. | ID | Opposite wood | Tension wood | OW/TW |
CF234721 | Caffeic acid 3-O-methyltransferase | 5008 | 3511 | 1.43 |
CF227888 | 4-Coumarate-CoA ligase | 3574 | 1926 | 1.86 |
CF231217 | Peroxidase | 3566 | 3083 | 1.16 |
CF231974 | Phenylalanine ammonia-lyase | 3078 | 806 | 3.82 |
CF234324 | Ferulate-5-hydroxylase | 2731 | 3668 | 0.74 |
CF237253 | Phenylalanine ammonia-lyase | 2638 | 1192 | 2.21 |
CF232111 | Cinnamyl-alcohol dehydrogenase | 2638 | 3805 | 0.69 |
CF234410 | Caffeoyl-CoA O-methyltransferase | 1289 | 844 | 1.53 |
CF232637 | Phenylalanine ammonia-lyase | 716 | 569 | 1.26 |
CF235645 | Laccase | 632 | 530 | 1.19 |
CF230510 | Peroxidase | 467 | 429 | 1.09 |
CF228407 | trans-Cinnamate 4-monooxygenase | 426 | 451 | 0.94 |
CF236951 | Ferulate-5-hydroxylase | 294 | 334 | 0.88 |
CF227438 | trans-Cinnamate 4-monooxygenase | 250 | 208 | 1.20 |
CF235596 | Peroxidase | 122 | 92 | 1.33 |
CF231337 | Peroxidase | 33 | 67 | 0.49 |
CF235789 | Cinnamoyl CoA reductase | 23 | 9 | 2.56 |
CF233986 | 4-coumarate-CoA ligase | 16 | 7 | 2.29 |
CF227399 | Phenylalanine ammonia-lyase | 13 | 7 | 1.86 |
4.4 Genetic engineering
The availability of accurate microscopy techniques based on immunochemistry will make it possible to define precisely in which cells of the wood and even where in these cells the proteins encoded by genes regulated in tension wood are localised. Moreover, the assessment of transgenic trees for their ability to differentiate tension wood will certainly allow for unravelling the function of unknown proteins expressed in tension wood.
Transgenic trees (mostly poplar trees) targeted for nearly all the different lignin biosynthetic genes have already been produced. These trees exhibit a wide array of modifications at the level of the lignin polymer (see [54] for a recent review). The evaluation of these transgenic trees for potential alterations in their ability to form tension wood may shade some light on potential causal links between lignin characteristics and cell-wall mechanical behaviour. For example, it has been demonstrated in 4CL down-regulated poplars that a repression of lignin biosynthesis promotes cellulose accumulation [52]. It remains to determine whether this strong interrelation occurs at a metabolic level through carbon reallocation or at a developmental level through a delayed xylem differentiation and prolonged cellulose deposition.
CAD down-regulated transgenic poplar trees are characterised by a strong red coloration in the differentiating xylem [55] (Fig. 3). Interestingly, it appeared that this red coloration is absent from tension wood formed in bent stems (Photo 3). Although the nature of the compound responsible for this coloration is still not elucidated, it is likely incorporated in the lignin polymer. If this is the case, one may wonder why the red coloration seems absent from tension wood. Upon gravity induction, several other transgenic poplar trees with much reduced levels of CAD activity [56] seemed altered in their ability to form gelatinous fibres (Pilate et al., paper in preparation). This suggests a causal relationship between lignification and G-layer differentiation. Such transgenic trees certainly constitute a precious material to assess the potential function of lignin in tree physiology and in particular in the generation of growth stress. Using integrative approach, associating molecular biology, biochemistry, anatomy and biomechanics, we may expect that the function of lignification in tension wood formation will be in the near future better understood through the evaluation of the ability of these different transgenic trees to form tension wood.
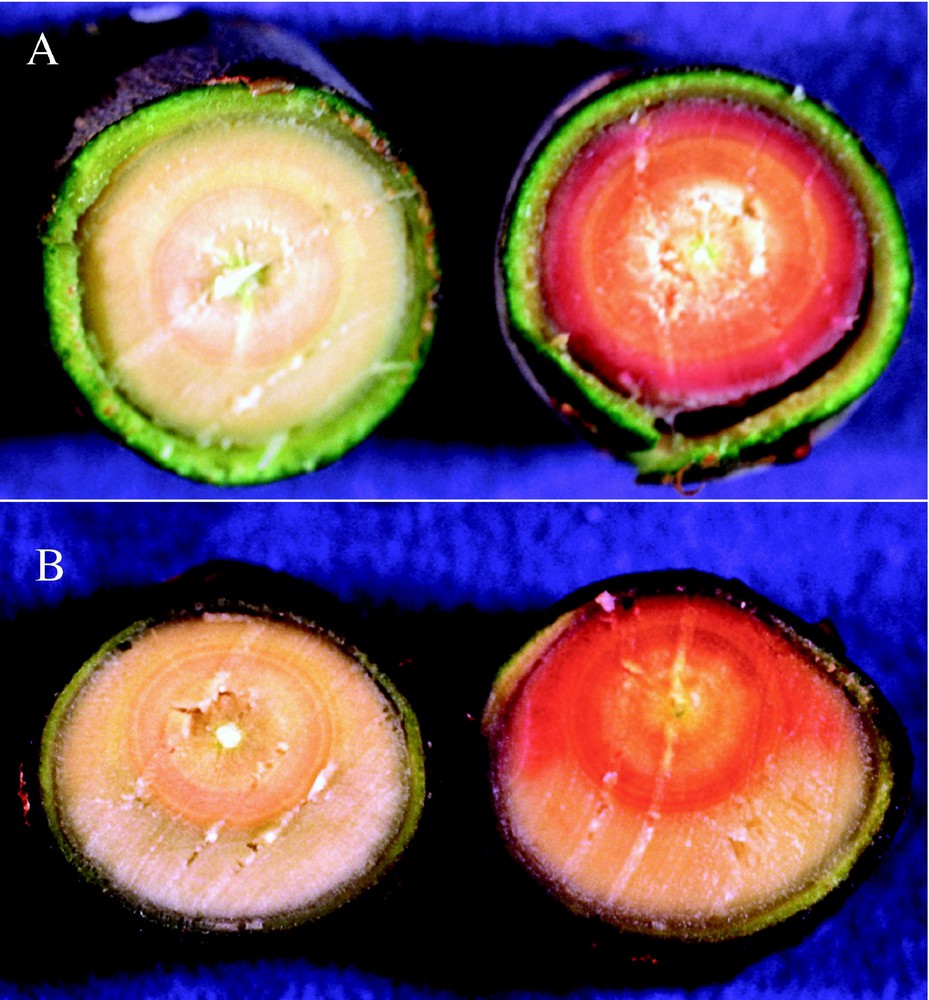
Section of wild type or transgenic poplar kept straight (A) or bent (B). Note the excentricity and the crescent of tension wood in the bent stems. Transgenic poplars exhibit a red coloration in the differentiating xylem characteristic of low CAD activity. Interestingly, the tension wood area from bent trees is devoid of this coloration.
4.5 When genomic meets with physiology
Previous biochemical observations have been confirmed by genomic studies: this is the case for lignin and cellulose biosynthesis during tension wood formation. Genomic studies have also allowed the identification of genes and proteins potentially involved in tension wood formation. This is the case for AGP and also for several other genes of unknown function that were highly expressed in tension wood. In the future, we can anticipate that our knowledge on different aspects of tension wood formation will increase: this is already the case for the role of plant-growth regulators. Indeed, redistribution of growth regulators is thought to be involved in the transduction of the stimulus responsible for the induction of reaction wood formation [57]. For a long time, auxin (indole acetic acid) was the preferred candidate. However, quantification of endogenous auxin levels in poplar stems induced to differentiate tension wood leads to the conclusion that auxin distribution is most likely not the primary factor [58]. Ethylene appears now as a strong candidate. Indeed, Andersson-Gunnerås et al. [50] have recently demonstrated that ethylene production occurs asymmetrically within poplar stem upon induction by a gravitational stimulation and that a gene coding for ACC oxidase is strongly regulated in tension wood. It is worth reminding here the existence of a link between ethylene and lignification as evidenced in the phenotype of an Arabidopsis mutant [59], where the mutation of a chitinase-like gene causes an ectopic expression of CCoAOMT, an ectopic deposition of lignin and an overproduction of ethylene. Moreover, the mutation affected some aspects of plant development including an exaggerated hook curvature. It remains to determine if such a relation between lignification and ethylene also exists in the stem of a tree subjected to a mechanical stress. The endogenous substrate of chitinase-like genes in plant is still a matter of debate: interestingly, chitinase has been shown to be necessary for somatic embryo development [60], which is also controlled by AGP [61]. The possibility that certain AGP contain N-acetyl-glucosamine residues that may be processed by chitinase to produce a cellular signal certainly deserves further examination. Further studies are certainly needed to assess any potential relations during tension wood formation between lignification, ethylene production, chitinase activity, and AGP.
5 Conclusion
For several decades, a lot of studies have been carried out in order to elucidate what leads to tension wood formation and stem reorientation in hardwood trees. The accumulation of data and the building of models have made possible to increase our knowledge on this physiological adaptation of trees to their environment. With respect to lignin formation, it seems probable that the lignin differences (in amount, composition and structure) between the different layers of tension wood fibres have a function in the ability of tension wood to reorient stems or branches toward their equilibrium position. Obviously, there is still a lot to do in order to better know how lignin and lignification are involved in the definition of tension wood mechanical properties. With the refinement of lignin localisation techniques and the development of functional genomic approach, we may expect in a near future that important progresses will be made in our knowledge on tension wood formation and more particularly on the function of lignin and also of other molecules such as AGP. The new data that will be generated using these novel techniques will help to refine and validate existing models.
Acknowledgments
Several data have been produced within the Agrice project Plantes ligno-cellulosiques et herbacées : modulation de leur lignification et propriétés d'usage par génie génétique. SEM photos have been shot at the ‘Centre de microscopie électronique de l'université Claude-Bernard, Lyon-1’.