1 Introduction
As is the case for all species cultivated for their seeds, the final seed yield in grain legumes (namely the weight of seeds produced per area unit) is highly correlated with variations in the number of seeds produced per unit area. Nevertheless, the variability of individual seed weight at harvest can still account for the residual variability of yield (approximately 20%) that remains unexplained by the variations in number of seeds. Moreover, seed protein content is a major quality criterion for the commercial value of legume seeds. Seed protein concentration at harvest depends on the differential supply of carbon and nitrogen during the seed filling period. Indeed, legume seeds store large quantities of storage proteins to support germination and seedling emergence (for reviews, see [1] and [2] in this issue). Legume seeds, notably from pea (Pisum sativum L.), are large, rich in protein, and thereby widely used as protein sources for human and animal consumption. Consequently, the ability of the pea crop to accumulate carbon and nitrogen reserves within its seeds is a major component of seed yield and quality of harvested seeds.
Understanding the mechanisms of yield formation throughout the plant growth cycle requires an analysis of individual seed weights. The indeterminate growth habit of legume plants is characterized by a significant period during which seed set and seed filling occur simultaneously. During this period, young pods that bear seeds still susceptible of aborting compete for assimilates with both vegetative growth and older pods of which seeds may have begun accumulating reserves in their cotyledons. Therefore, the establishment of the seed number per node along the stem (called the “seed number profile”) depends upon the demand for assimilates of growing seeds that already developed at the lower nodes (a node defines the place on a plant stem where a leaf is attached).
Individual seed weight is the product of individual seed growth rate and the duration of seed filling. In grain legumes genotypic variation in individual seed weights is mainly due to differences in individual seed growth rates, the large-seeded genotypes exhibiting the highest individual seed growth rates [3–5]. Variations in the duration of seed filling among genotypes and environmental conditions have also been reported [6,7]. However, for a given genotype, variations in individual seed weights among different environmental situations are mainly due to variations in seed growth rates, even if the duration of seed filling varies [5,6].
2 Seed development
Seed developmental stages are similar among grain legume species. Following fertilization, seed development can be divided into three developmental phases. i) A first reproductive stage begins at fertilization and ends at the “beginning of seed filling”. Here, cell divisions take place in the embryos without significant accumulation of dry matter, and seed water concentration is stable at approximately 85% [8]. At the end of this phase, seeds are unlikely to abort [9] and both starch and protein reserves start accumulating within the cotyledons; at this stage the pod wall reaches approximately its final size [10]. This stage is referred to as the “beginning of seed filling” and corresponds to the final stage in seed abortion. For pea, this first phase lasts approximately 300 degree days (0 °C being the base temperature, that is the lowest T at which plant growth can occur). ii) A second phase begins at the stage “beginning of seed filling” and ends at physiological maturity. Because seed coat expansion is almost completed when the accumulation of starch and proteins begins [11], seed weight at the beginning of seed filling corresponds approximately to the weight of the seed coat [12]. During this second phase, cell divisions stop, while near-linear dry matter accumulation starts in cotyledons and continues until physiological maturity [8]. Seed water concentration decreases regularly until a seed water concentration specific of the considered species [13–15]. When this specific seed water concentration is reached [55%, 60% and 60% for pea, soybean (Glycine max) and white lupin (Lupinus albus L.), respectively], vascular connections between developing seeds and the mother plant are disrupted, corresponding to physiological maturity. iii) After physiological maturity, the final third phase corresponds to seed desiccation (for a review, see [16] in this issue) during which the mature seeds passively lose their water at a rate depending on environmental conditions (i.e., ambient air humidity) [14].
Seed water concentration is a convenient indicator of these developmental stages [17], as it is specific to each stage and stable for a given genotype among various environmental conditions [13–15].
3 Determination of individual seed growth rate
Studies on the effect of variations in assimilate availability during the filling period on seed growth of species with an indeterminate growth habit often lead to contradictory results [18,19]. Because the seeds located along the stems of a same plant can reach different developmental stages at a given time, changes in assimilate availability during the filling period cannot be analyzed at the whole plant level but should take into account the precise developmental stage reached by each of the developing seeds.
3.1 Seed growth rate is highly correlated to the number of cotyledonary cells
At the whole plant level, modifications in photoassimilate availability do not affect seed growth rate if they occur after the completion of embryo cell division [20]. A reduction of assimilate availability does not entail a decreased seed growth rate, whereas total plant growth and duration of seed filling are reduced. In the same way, an increase of assimilate availability does not lead to any increase of seed growth rate, whereas total plant growth and duration of seed filling increase. Hence seeds have priority for assimilates over both vegetative growth and seed formation in the upper part of the plant. Because the flux of assimilates in growing seeds is rather stable it can be assumed that seed growth rate remains constant during the seed filling period, meaning that individual seed growth rates at the beginning of seed filling reflect the potential individual seed growth rates. Because cell divisions cease at the beginning of seed filling, the cotyledon cell number is fixed. A relationship between cotyledon cell number and seed growth rate has been established in pea and soybean (Fig. 1) [21]. Consequently, seed growth rate observed during the seed filling period is determined before this period, during cell division in the embryo.
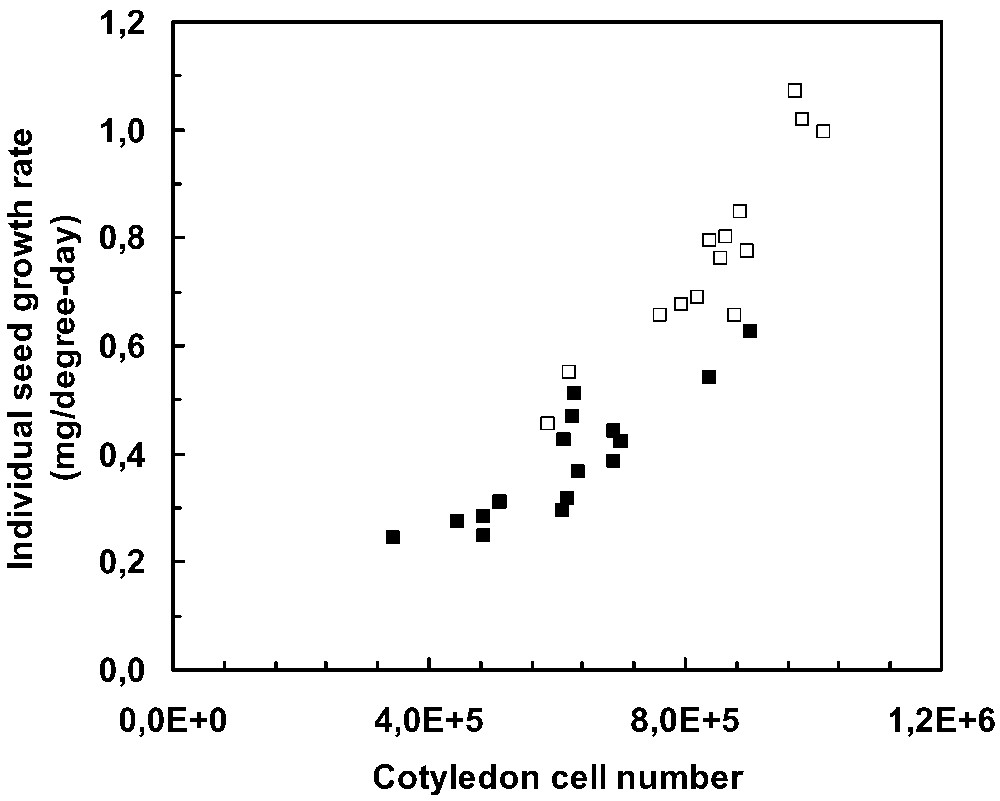
Relationship between seed growth rate and cotyledon cell number. Pea: Solara, large-seeded genotype (□); Frisson, small-seeded genotype (■) [21].
For various experiments conducted under farming practices, the relationship between cotyledon cell number and seed growth rate is valid across genotypes differing in their seed size (Fig. 1). Because seed weight differences among genotypes are mainly due to differences in seed cell number, this suggests that the seed cell number is a major factor controlling seed growth rate and final seed weight. Furthermore, for a given genotype, differences in seed growth rate among morphological positions on the same plant and among differing environmental conditions are strongly and positively correlated with differences in seed cell number. Thus seeds located at the top of the plant have a higher cotyledon cell number than older seeds located at the base of the plant.
To conclude, seeds are a high priority sink in assimilate partitioning [22] and the potential individual seed growth rate is achieved regardless of the intraplant competition level during seed filling. The duration of seed growth is shortened only if photosynthetic activity is not sufficient to fulfill assimilate demand of filling seeds. Consequently, seed growth rate observed during seed filling period is determined during the cell division phase in the embryo: variations of seed growth rate depend on growing conditions during the period between fertilization and beginning of seed filling that affect seed cell number.
3.2 Variations in seed cell number
The duration of the cell division period of seeds located in the upper part of the plant is shorter than that of older seeds located in the lower part of the plant [13]. As seeds borne by reproductive nodes located at the top of the plant have a higher individual seed weight (i.e., they have a larger number of cells) than those of the first reproductive nodes (lower parts of the stem), this signifies that mitotic activity increases with the position of the reproductive node, from the base to the top of the plant.
The variations of mitotic activity in seeds as a function of the nodal position is partly linked to the sucrose delivered to the embryos during cell division. The sucrose concentration in phloem sap supplied to the seed at the upper nodes is higher than that at lower nodes. Such an increase may be a consequence of xylem-to-phloem transfer [23,24] and/or enhanced contribution of neighboring leaves to the carbon economy of the closest fruits [25], especially in the upper part of the plant intercepting the major part of solar radiation for the whole plant. A relationship between seed growth rate and sucrose flux per seed suggests that the flow of sucrose delivered to the seeds during the cell division period is an important determinant of seed growth potential [26].
As seed growth rate is highly correlated with the number of cotyledonary cells produced during the cell division period, individual seed growth rates might be considered as a convenient indicator of cell division between the beginning of flowering and the beginning of seed filling. Indeed, studies using isotopic labeling of the sap flow and simultaneous measurement of seed embryo mitotic activity showed that sucrose is instrumental in seed embryo mitotic activity modulation. Knowledge concerning the plant cell cycle regulation has been gathered and provides a mechanistic explanation. Sucrose modulates cyclin expression, which is involved in the regulation of the cell cycle [27]. Moreover the cotyledon cell number at the end of the cell division period is correlated to the hexose concentration in the apoplasm (within a plant, the apoplast is the free diffusional space outside the plasma membrane) and to the activity of an acid invertase involved in the cleavage of sucrose into hexoses [28].
Even if sucrose flux in phloem sap is proposed to be an important determinant of mitotic activity in seed embryo, mitotic activity should be more thoroughly investigated especially in relation the environmental factors, especially such as temperature [3].
3.3 Variability of the duration of the cell division period in the developing seed
There are only few studies dealing with the variability of the duration of the seed cell division period under field conditions. For a given genotype in optimal growing conditions, this duration is likely to be constant when expressed in degree-days [29]. However, this optimal duration is also under genotypic control [13], being higher for large-seeded genotypes and could be under the control of the embryo [30]. Moreover, data obtained for field-grown plants subjected to either water, thermal, or nitrogen stress indicate that for a given plant node the duration between flowering and the beginning of seed filling, which corresponds to the cell division period, could be reduced under stress conditions [31,32]. Consequently the duration of cell division is longer when assimilates availability is high than under stress conditions.
4 Determination of the duration of seed filling
The accumulation of reserve compounds in seeds during the seed filling period depends on their photoassimilate demand, which is determined by their number of cotyledon cells [21]. Fulfillment of this demand depends on both the instantaneous carbon and nitrogen assimilation by the plant, and on nitrogen remobilization accumulated in the plant before the seed filling period [33]. Consequently, the duration of seed filling at a given node is likely to be affected by assimilate availability [20,34]. Two mechanisms can lead to the termination of seed filling, depending on the source-sink ratio during the filling period. i) In most agricultural situations a self-destructive process caused by nitrogen remobilization to filling seeds terminates reserve accumulation, corresponding to monocarpic senescence [35]. Here, the high nitrogen demand of the seeds triggers the mobilization of proteins involved in photosynthesis (as Rubisco) [36], which entails a stop in the production of photoassimilates needed for seed growth. ii) However, in a few instances seeds reach their maturity even though nitrogen available for remobilization to filling seeds has not been exhausted. In such cases where self-destructive processes are less intensive, carbohydrates could still be synthesized in leaves and thus termination of seed filling then occurs because the seeds have reached their maximal seed size.
4.1 Intrinsic limitation: maximal seed weight
The maximal seed weight is the product of the cotyledon cell number and the maximal cell size [3]. The determinism of the cotyledonary cell number has been presented above (§3.2). Therefore, this paragraph on the intrinsic limitation of seed weight will only deal with variations of the maximal cotyledonary cell size.
4.1.1 Genotypic effects
As for many species, the endoreduplication process (whereby chromosomes duplicate without any division of either the nucleus or the cell) occurs in cotyledons after the beginning of seed filling in pea [37]. For seeds from several genotypes or seeds obtained by reciprocal crosses, grown without assimilate limitation, the mean volume of the cell cotyledons is highly positively correlated with the endoreduplication level in the mature cotyledon [30]. Thus, the control of the maximal cell size could be linked partially to the endoreduplication cycles in the cotyledonary cell.
4.1.2 Environmental effects
For a given seed cell number, the higher the temperature of the pod during the seed filling period the smaller the mean maximal cell volume (Boutin and Munier-Jolain, unpublished data). Consequently, the mean maximal cell volume depends on the environmental conditions during seed filling. For those experiments the duration of seed filling expressed in degree-days decreases concomitantly with the elevation of temperature. It can be concluded that the maximal duration of seed filling cannot be considered as a genotypic constant.
4.2 Exhaustion of carbon and nitrogen resources
Although the intrinsic limitation of seed weight may be responsible for the termination of seed filling, the exhaustion of plant protein reserves represents the most encountered cause of seed-filling ending in field [38]. Synthesis of reserve compounds in pea seeds (starch ≈51%; proteins ≈24%; [39]) depends not only on the instantaneous supply of carbon and nitrogen, but also on remobilization of nitrogen previously accumulated in vegetative parts. During the seed filling period, carbon supply depends mostly on current photosynthesis [40]. Conversely, nitrogen newly acquired during the seed filling period is unlikely to fulfill the high nitrogen demand of filling seeds, because both soil nitrate assimilation and nitrogen fixation decline during this period [41]. Thus, nitrogen accumulation in seeds becomes metabolically closely associated with nitrogen remobilization from the vegetative parts of the plant. Nitrogen remobilization affects several nitrogen pools in vegetative parts [36,42]. Rubisco, which is required for CO2 fixation, constitutes a significant nitrogen source for remobilization as it the most abundant protein on the planet, representing about 50% of the total foliar proteins [43]. Proteolysis and the export of nitrogen compounds lead to a decrease in the leaf nitrogen content per unit of leaf area, which affects the leaf photosynthetic activity [44]. The reduction of photosynthetic capacity triggered by protein mobilization brings about the end of the seed filling period, when the plant carbon supply can no longer fulfill the seeds' demand.
4.2.1 Characterization of the amount of nitrogen available to filling seeds
As stated above, nitrogen accumulated in seeds during the seed filling period has two origins: the exogenous N being assimilated and/or fixed by the plant and the remobilized N from N source organs. The N accumulated by the plant during the seed filling period significantly contributes to the total N accumulated in seeds at harvest. Thus, 25 to 40% of the total amount of N in plants can be accumulated during the seed filling period [45,46]. In legume plants, nitrogen nutrition during the seed filling relies on both symbiotic fixation and soil mineral N absorption. Even though symbiotic fixation decreases during the seed filling period, it seems to be still effective and to stop only at the physiological maturity stage [47,48]. When mineral N is still available in soil during seed filling period, soil mineral N absorption can continue and contribute to seed nitrogen accumulation [48].
Remobilization processes involve both N accumulated by the plant before the beginning of seed filling and exogenous N accumulated by the plant during the seed filling period. Remobilized N from N accumulated in N source organs before the beginning of seed filling contributes to about 60% of the final N yield. However, 15N labeling of the exogenous N accumulation pathway has shown that nearly 90% of the N that was accumulated in seeds arose from N remobilization from the other plant parts [49].
The major part of the N accumulated by the plant during the seed filling period is allocated to seeds either directly or after transfer via vegetative parts [49]. The physiological mechanisms controlling nitrogen fluxes in pea plants are most probably very complex. The transfer of N accumulated by the vegetative parts to the seeds seems to be a slow process [50]. Thus, the N accumulated by the plant does not seem to be immediately available to the seeds, but rather to be invested in vegetative compartments that would then allow, after protein degradation in vegetative parts [36], the release of an equivalent N amount to the seeds. Leaves and pods are the major contributors to the N supply of seeds while stems and roots were shown to only provide approximately 10% of the N remobilized to the seeds [49]. However, a proportion of N in the source organs is not available for remobilization to the seeds because it is associated to parietal structures [51].
4.2.2 Influence of the temperature during the seed filling on the amount of N available to seeds
The temperature during the seed filling period may influence plant N partitioning [52]. The scarce results available for pea and soybean suggest that neither exogenous N accumulation nor the rates of N remobilization are affected by temperature variations across a moderate temperature range of 13–29 °C [53]. However, competition between seeds and vegetative sinks could be modified when temperature is lowered while solar radiation is maintained, as a consequence of an increase in plant carbon accumulation when expressed in degree-days. The resulting additional C flux can allow new competing vegetative sinks to grow and the increase in vegetative growth seems to attract a part of available N at the expense of filling seeds [53]. Thus, seed N concentration decreases when air temperature decreases during the seed filling period, reflecting a reduction in N availability to filling seeds due to the formation of new competing sinks.
4.2.3 Determinism of the individual seed N accumulation rate
The amount of N potentially available to filling seeds at a given time can be estimated using the amount of exogenous N accumulated by the plant and the amount of remobilizable N. The characterization of the amount of N available to filling seeds allowed establishing a positive relationship between the rate of individual seed N accumulation and plant N availability [33]. However when plants are grown without assimilate limitation, the rate of individual seed N accumulation reaches a maximum rate of N accumulation, which cannot be exceeded in spite of large N availability. This maximum rate of individual seed N accumulation varies among genotypes in correlation with the individual seed weight [54]. It demonstrates that the individual seed N accumulation rate increases with an increase in plant N availability until a maximum rate of individual seed N accumulation.
5 Concluding remarks
The present knowledge on the rate of seed N accumulation has been synthesized in a model simulating N partitioning to seeds during the seed filling period in pea [54]. This model, which was conceived to be introduced in a global model of pea crop functioning, will be an important component to simulate seed protein concentration, N concentration in vegetative parts and the date of termination of seed filling for this plant. In the same way, knowledge concerning the processes involved in variations of individual seed weight, and their interaction with environmental conditions, could be included in a model simulating the potential seed size. Such models are useful to dissect the phenotypic characters impacting seed protein content and productivity and their genetic variability [55]. Because they allow identifying the respective effects of environment, genotype and their interaction on phenotypic characters they constitute useful tools to improve agricultural practices to manage fluctuating environments that have main impacts on yield variability, or plant breeding. In the future improving seed production, but also seed protein content, especially the sulfur-amino acid content in seeds will help to develop legume production in Europe.
Acknowledgements
The authors thank UNIP (Union National interprofessionnelle des plantes riches en protéines) who funds a large part of this work.