1 Introduction
In a series of articles [1–4], we have proposed that morphogenesis of avian embryos may be amenable to a physically reductionist theory, by which a hyperbolic viscoelastic tissue flow would transform a round piece of tissue (the initial animal “blastula”) into a recognizable vertebrate.
To summarize: when the morphogenetic movements start, the embryo is round, but later when gastrulation begins the tissue flow on the ectoderm shows a broken symmetry so that its pattern (the vector field) in biomechanical terms can be described as a hyperbolic, bidirectional flow away from a stagnation point (Fig. 1). In the reference frame of the stagnation point, the tissue flow winds away towards the cranial and caudal ends of the embryo, avoiding the stagnation point, which, for reasons explained below, will eventually become the area of the yolk sac stalk in avian embryos. We propose that the broken symmetry of the flow is progressively up-scaled to the adult animal pattern, in such a way that the areas with straightforward tissue movement are the ones where axial structure will develop, and the four lateral quadrants of the windings of the tissue colocalize with the future limb plates. Interestingly, this broken symmetry is characterized by a rotation of the tissue with a chirality of opposite signs on the left and right sides of the embryo, and on the anterior and posterior sides of the embryo; this may explain readily the opposite chiralities of the right/left and upper/lower extremity patterning.

Here we overlay an ideal hyperbolic flow, over a chicken embryo image, at the 14 somite pairs stage (HH 11–12). The limb buds are already visible, as faint tissue bumps. The flow pattern is bidirectional away from a stagnation point, it is convergent towards the median axis, and divergent along the median axis. The trajectories form hyperbolic lines.
Whether this is general to all tetrapods will deserve a detailed case study of many vertebrate taxons. However, a direct dorsal observation of an adult animal, for example a cat, or embryos of other classes of vertebrates, e.g. a direct frog (courtesy of Prof. Richardson), seems to confirm this fact (Supplementary Fig. 1).
The existence of the stagnation point is not a trivial fact as it may not be shifted to another place by changing the reference frame. Indeed, embryogenesis occurs inside an egg which is an absolute frame of reference for morphogenesis. In this reference frame, the average force, and average movement is zero. Inside this egg, morphogenetic movements occur by mechanical forces. While the embryo can be translated (gedanken experiment) uniformly by any Galilean transformation (e.g.: eggs could be cultured on board of a car), the transformation of the reference frames associated to other parts of the embryo are not Galilean, because their speed is not constant. The hyperbolic point of the embryo has a constant Galilean speed with respect to the egg reference frame, since it is zero.
A paleontologist, Jeffries Wyman, had a similar intuition about the existence of this hyperbolic flow in vertebrate embryos already in 1868 [5], but was unable to demonstrate it, although he did review a significant number of animal vertebrate taxons. It is not surprising that this view has progressively disappeared. Also, already in 1924, the work of Wetzel shows a hyperbolic point at the early blastula stage [6].
The proposed theory adds a new powerful factor that can act both as a trigger that launches the patterning processes and/or guide them throughout the development. This fills in gaps in the current genetic induction models, by explaining why similar genetic pathways lead to a globally organized pattern with internal broken symmetries, which are not present in the genes themselves. Indeed, when taking into account that all genetic pools in the embryo are being advected in the presence of such a hyperbolic flow, it is logical to assume that the appendages or body parts that are induced by those genetic pools will at the same time inherit the asymmetry from the flow. Surprisingly enough, this idea has met with a considerable violence in the field of developmental biology [7].
The first objective of this article is to provide the experimental evidence that early embryonic material is viscoelastic. This will be obtained by air puff tonometry [4]. The second objective is to show a detailed map of the tissue flow during the vertebrate embryo morphogenesis and demonstrate that the embryo tissue passes through crucial stages when a hyperbolic flow is evidenced. Thirdly, we will correlate this flow to actual morphogenetic events that will result in formation of body parts which will eventually become visible on the adult animal. This is to say that we will show that the broken symmetry of the initial flow, appearing at the blastula stage when the animal is formless, has global properties which are transmitted progressively, as the animal grows, to the upper scales of the animal form itself. Stated otherwise: a property of the flow map inside a flat and formless animal (hyperbolic pattern) becomes a property of the morphology of the animal itself, by viscoelastic deformation of the embryonic tissue [2]. The animal model is the chicken embryo, because it is almost flat at early stages and allows precise recording of the vector fields of the tissue flow.
Indeed, by a careful observation of tissue movements at all the developmental stages between the early gastrula stage and the first signs of limb outgrowth, and with both top and bottom views, we will demonstrate below that the animal pattern is formed by the viscoelastic deformation of the tissue in a bidirectional hyperbolic flow. The broken symmetry of the flow is progressively up-scaled in the adult animal pattern (Supplementary Fig. 1), following the principle of Curie [8].
We will show that the ectoderm of the developing embryo rotates rostrally and caudally away from a hyperbolic point located exactly at the (future) yolk sac stalk area (which is expected to correspond to the umbilical area of mammals1). The axial structures are formed along the midline in the areas with a strong bidirectional straightforward flow. During this process, most of the somites (precursors of several tissues, including vertebrae tissues, albeit with a shift in wavelength), do not drift from head to tail, as generally thought, but from the fixed point “upwards”. This is to say that the somites are indeed formed in an Anterior to Posterior direction, but they move in the opposite direction; this forms a gust of somites moving anteriorily. Four extremities with opposite chiralities originate from the regions of vortex–type flow in the lateral areas above and below the fixed point. Thus being a tetrapod is not a matter of chance, but the attractor of such a tissue flow. We will also demonstrate that the flow contributes to prepatterning of the vascular bed of the (presumptive) yolk sac stalk region of the chicken embryo and generates the tubular structures of the heart and major blood vessels.
Thus, the flow contributes greatly to establishment of the entire body plan. This means that there exist no “presumptive territories”: instead, the dynamics of the tissue establishes them.
2 Methods
Chicken embryos are incubated in a standard Minitüb incubator, maintained at 38 °C. After 1½–2½ days of incubation, each embryo is extracted out of the egg with extreme care and laid flat on a glass Petri dish with approx. 5 mm deep of PBS buffer. The Petri dish is gently stirred by hand, and all the yolk is progressively removed. The vitelline membrane is removed as well. The embryo is then put to incubate on a flat plate at 37 °C with a second glass plate put on top, at 38.5 °C, the temperature difference between the two plates being crucial for condensation preventing. In such conditions, an embryo survives and develops for up to 10 hours normally.
Stacks of images of embryos at different stages of development are obtained during the time periods of up to 5 hours, with a time resolution of 30 seconds using grazing illumination with a fiber lamp from Schott, Leica MZFlIII upright microscope, and a B&W Watek 512 analog camera interfaced with the Scion Image software. For a few movies, a double system of imaging was used, allowing one to film the embryo from the top, and from underneath using grazing illumination with a fiber lamp from Schott, the upright microscope is used for the top view and an Optem zoom for the underneath view, and 2 B&W Watek 512 analog camera interfaced with the Scion Image software. In this case, a film composed of two views of the same embryo, is generated, and the time interval is 1 minute (see, for example, Movies 6,7).
Image processing included correction of the sample drift by Stackreg plugin of the ImageJ software. Once the stacks are registered, the vector field of the flow is extracted by Particle Image Velocimetry (PIV) tracker module (ImageJ plugin developed by O. Cardoso). All movies are freely accessible at the address: http://www.msc.univ-paris-diderot.fr/∼vfleury/portailembryons0.html.
3 Air puff tonometry
In order to analyze the viscoelastic properties of embryonic tissue, we have developed a new instrument, namely a Scanning Air Puff Tonometer (SAPT). The detail of the instrument is presented in [4]. It is an evolved version of the air puff tonometer used classically, and even routinely, by optometrists to measure the mechanical properties of the cornea, in the context of Intra-Ocular Pressure (glaucoma). In the prototype used here, a short and tiny air puff is blown through a glass micropipette constantly, in order to see what is the reaction of the surface (deformation), and deduce its physical (material) properties. In the version used for the data presented here, an ultrarapid camera Photron Fastcam was used, instead of the classical video B&W camera of [3]. The air puff corresponded to a flow of 2 cm3/min through a pipette of diameter 20 micrometers. This corresponds to a total force equal to ∼10−7 Newton. When the air puff occurs, the surface of the embryo tissue is deformed by the air shot, and the specular reflexion appears dark over a brighter background (shadowgraphic image).
Also, we measured the absolute depth of the “hole” area during time, and also the thickness of the limb plate, by laser profilometry (data not shown). The laser profilometry is performed with a glass fiber introduced inside the micropitette used for the air shot [4]. This shines a light spot at the point under study. During the air puff, the displacement of the spot allows one to measure the absolute depth of the indentation. Also, the same light spot is used to measure the height of the lateral plate, by moving the embryo at rest (no air puff) along one direction perpendicular to the limb plate. The absolute height of the limb plates for the embryo in Fig. 2 was found to be 60 micrometers. The deformation observed during the air shot was of the order of 20 micrometers. Therefore, the maximal relative deformation which was generated was of order 0.3.

Left: Direct view of the embryo at the early recession of Hensen's node. Right: the surface response to air puff tonometry performed through a pipette (diam. 20 μm) shows a viscous creep after a few seconds. More examples are to be found in Supplementary Fig. 2.
The time transient gives the viscoelastic time scale of the tissue, the asymptotic deformation rate gives the viscous behavior. If the tissue has a viscous component, the deformation should asymptote a constant deformation rate as a function of time, for a constant force. This is at variance with say, the cornea measurement of optometrists. In the latter case, a constant deformation is reached after the transient, corresponding to a saturation of the deformation. This saturation corresponds to the permanent elastic deformation under a constant stress. For a tissue to behave as a fluid, a constant deformation rate must be reached asymptotically, not a constant deformation.
4 Results
4.1 Results of air puff tonometry
The air puff tonometry which was performed from the early gastrula stage onwards, shows a linear deformation rate, after a rapid viscoelastic transient (Fig. 2 and Supplementary Fig. 2). For the Figure, we give the global image, the shadowgraph image during the air puff, and the response of the surface analyzed by shadowgraph. The plot represents the evolution of the radius of the indentation, in the presence of a constant air puff. The graph in Fig. 2 is during the notochord extension and early neurulation; a similar behaviour was obtained at primitive streak (PS) stage and at the 10 somites stage. A deformation rate was found asymptotically. The fact that the indentation asymptotes a straight line of constant slope vs time shows the behaviour of a viscoelastic material. The rapid transient corresponds to the viscoelastic response of the material, and the eventual constant slope of the displacement vs time corresponds to the slow viscous creep, found in the long term. The data actually plots the variation in time of the cavity radius, we assume however, that the radius of the cavity varies like its depth, and knowing the absolute thickness (∼60 μm), the data are proportional to deformation rates. For times scales larger than a characteristic time of 4 s, the embryonic material has a viscous behaviour.
This demonstrates that at such an early stage, the embryonic tissue is in fact a paste with a very simple viscous behaviour. Therefore, the speeds of enlargement of the observed air impact, are viscous speeds, and embryo morphogenesis is indeed a flow. The pattern of velocities which will be extracted now from the time-lapse movies are therefore technically fluid speeds.
4.2 Results from time-lapse movies
Fig. 3, Movie 1 presents the recording of the ectoderm tissue flow occurring at the time when the notochord starts to extend. It is clearly seen that the cellular movements upon the flat early gastrula consist of a rostral and caudal tissue winding (Fig. 3(A)). Existence of the rostral vortices has been shown in [6], whereas to the best of our knowledge, it is the first experimental evidence of presence of another pair of vortices, caudal, where the orientation of flow has a completely opposite chirality. The stagnation point of the flow is located at the apex of the PS. The Movie 1, analyzed in Fig. 3(B), shows that the caudal winding is associated with the closure of the PS along the median axis. Prior to closure (Fig. 3B 0–100′), there is a pronounced flow component that contributes towards “invagination” of the tissue towards the median axis. The movement towards the median axis leads to closure “like a zipper” of the furrow along the median axis. Due to incompressibility of the tissues, the ectoderm that moves towards the midline of the embryo has no other possible direction of flow than winding caudally instead of diving (Fig. 3(B), 100′–150′). This flow results in a strong caudal pull of the ectoderm along the median axis, and brutal extension of the notochord. In the caudal direction the flow rotates laterally and forms two large caudal vortices that involve the ectoderm located at the lateral sides of the forming notochord. (Fig. 3(B), 150′–250′). By 3 hours after closure of the furrow, a hyperbolic winding is quite well established, by which the flow revolves towards the caudal area and cranial area, away from a stagnation point. As the chord becomes visible and the early signs of pelvic winding are seen, the hyperbolic flow is quite well seen (Fig. 3(C), Movie 2, stage immediately after 3(B)). The relationship between the furrow closure and the chord extension is obvious when following quantitatively reference points along the furrow (Fig. 4 top row), vs reference points in the area of Hensen's node. It is very clear that the invagination and the closure of the furrow provokes a recirculation of the tissue. The movement of the data points shows a falling to zero of the component of the speed oriented towards the furrow. Now, in the minutes following the closure of the streak, the tissue located in the area of the node undergoes a discontinuous stretch (Fig. 4 bottom row). This is characterized by a change in slope of the distance vs time plots. This demonstrates that the extension is correlated to the reshuffling of the physical forces due to tissue invagination, and migration of the mesoderm. Now, in order to confirm this, we have studied the mesoderm movement. This mesoderm movement is in itself a well documented fact [9,10], although how it correlates to the ectoderm movement is not so well understood. When the ectoderm invaginates through the furrow, a situation of tank-treading is created, with mesodermal and ectodermal layers crawling on each other in opposite directions. We have thus generated an underneath movie allowing us to follow a number of brighter spots of the mesoderm, as seen across the endoderm2 (Fig. 5, Movie 3). This shows that the mesoderm moves away from the streak, and gets almost arrested when it has filled the underneath cavity. At that moment, the streak is closed, and the ectoderm is stretched. Therefore, there is a very strong quantitative correlation between the physics of the mesoderm movement, inside its physical boundaries, the streak closure, and the ectoderm extension. The visual impression is that there is a moderate pull on the ectoderm (first part of movements in Fig. 4 (bottom), as long as the streak is open, and the mesoderm is poured into the underneath cavity. When the cavity is filled with mesoderm, the streak closes, and the ectoderm is stretched (second part of movement, after the arrows in Fig. 4(bottom), and 5. The rationale would be that while the mesoderm flows freely underneath, the ectoderm and mesoderm move in opposite directions. After reaching the physical boundaries of the cavity, the mesoderm is arrested by the contact to the boundaries, and its pull acts to move the ectoderm more strongly in the opposite direction, by the principle of action and reaction.
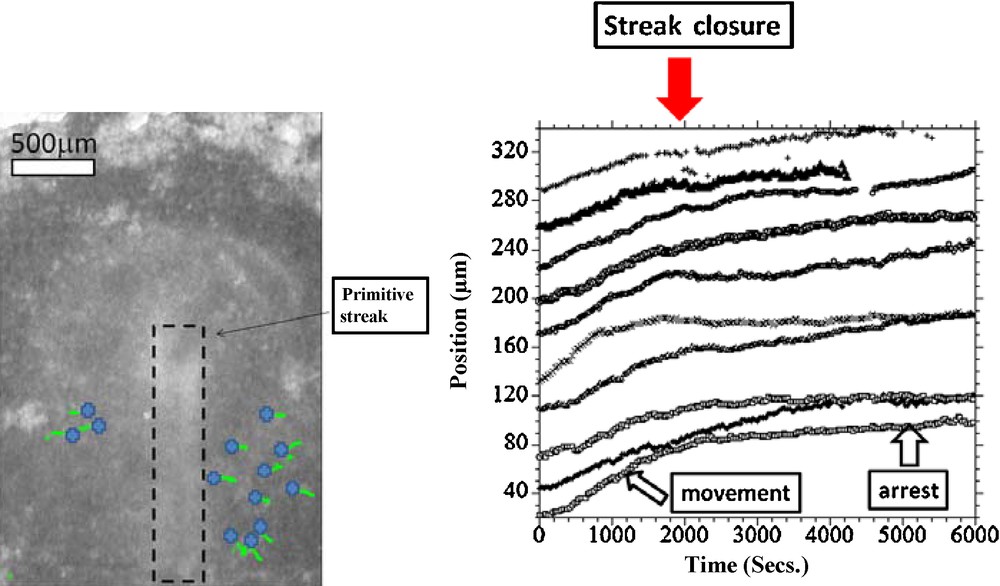
(Movie 3)Left: View of the embryo from underneath. The blue crosses represent the starting points for the PIV tracks. Right: The graph shows an analysis of the flow as observed from underneath, for a significant number of points selected manually as bright moving spots. We first evidence a flow away from the median axis, corresponding to the part of the tissue which has involuted and is now moving away from the median axis. The magnitude of the speed is ~1 μm.Min−1. For the sake of clarity, the curves were reset evenly along the Y axis, to avoid overlap. The points selected on either halves were translated and symmetrized to appear on the same graph. It is seen that the primitive streak closes (red arrow), at the moment when there is a rapid drop of the speed (slope of position/time), almost to zero. Closer to the median axis, the drop is almost discontinuous.
Next, as the embryo grows and reaches the 3 somites stage, the stagnation point remains, and the flow continues to revolve towards the head, and towards the tail (Supplementary Fig. 3, Movie 4). As the embryo reaches the 5 somite pairs stage, the tissue flattens along the body axis, but still, the windings oriented caudally and cranially, away from a point located in the presumptive yolk sac stalk area are clearly observed (Fig. 6(A), Movie 5). In the presumptive forelimb and hindlimb territories, vortex-like windings are very well seen which confirm previous indirect evidence [1,2]. There exists a hyperbolic point in the area of the presumptive yolk sac stalk. The tissue keeps expanding outwards of this point in both cranial and caudal directions (Fig. 6(B), and Supplementary Fig. 4). Also it is well seen that the somites do not drift from head to tail, as generally thought, but from the stagnation point “upwards”. (Supplementary Fig. 5 shows the drift of somites on typical embryos at two stages, 8 somites pairs, 13 somites pairs). It is indeed true that somites appear towards the posterior direction,3 giving the impression that they “descend”, but the entire frame in which they appear is moving anteriorily. This is to say that somite formation is a wave progressing on a tissue which moves anteriorily. This is why a large number of somites (about 20) will finally be found above the stagnation point, although the wave itself progresses posteriorily.
Thus, we have recorded the evolution of the tissue flow velocity map in developing chicken embryos starting from the time of notochord formation (HH 5 [11]) and up to 13 somites pairs stage (HH 12). Our data clearly confirm the existence of the hyperbolic bidirectional tissue flow, that appears slightly ahead of the anterior apex of the PS (Hensen's node region) and with a closure of the neural groove which migrates caudally, involving the entire lateral ectoderm in the areas of axial structure and limb plate formation (the groove progresses also cranially forming cephalic parts). The hyperbolic point of the flow is first located slightly ahead of Hensen's node region and in later stages finds itself in the presumptive yolk stalk area of the chicken (we expect similar effects for mammals, around the umbilical region).
The hyperbolic flow creates unequal physical conditions in the zones where major patterning processes are taking place during the studied stages or will occur later: (1) the area of the hyperbolic point, from where the tissue expands cranially and caudally, will become the yolk sac stalk (analog of the umbilical chord); (2) midline area with strong straightforward cranial/caudal tissue flow has patterning movements for axial structure formation; (3) the area lateral to the stagnation point will connect the blood system of the embryo to the yolk sac; (4) the two cranial and two caudal vortices with opposite chiralities in the lateral regions just above and below the yolk sac stalk will become the limb plates, where the limbs with opposite chiralities will eventually form. We propose that these unequal physical conditions, by affecting spatially the mechanoreceptor pathways [12] can be both the missing triggers (starting morphogenes) that initiate the patterning process, and the factors that modify locally the activity and effects of all morphogenes.
In the next part, we will focus on the effects of this hyperbolic flow on the circulatory system development.
The flow output towards the extraembryonic organs starts when the heart starts to beat, and blood (actually plasma) flow begins. At that moment, the presumptive umbilical region is determined for good. It is well-known that blood vessels develop by coalescence of blood islands formed by haemangioblasts [13]. Since the tissue flow winds in opposite directions above and below the presumptive yolk sac stalk area, the tissue lateral to the “umbilical” region converges towards the stalk (pseudo-navel area) in a “funnel”-shape fashion (Fig. 7, and Supplementary Figs. 6 and 7). At this stage of development, the early blood islands form a seemingly “random” distribution of blood pools, but careful observation of the blood islands in this region clearly show that, in fact, they follow large organized paths oriented towards the “navel”, directed by the hyperbolic tissue flow (Fig. 7, box). This confirms previous preliminary reports on this question [2]. Following tissue flow, the capillary lattice forms a funnel exactly at the location of the presumptive yolk stalk, preparing a prepattern for the more mature vitelline arteries and veins. These become more conspicuous as the blood flow enlarges them (Fig. 8 and Supp. Fig. 7).
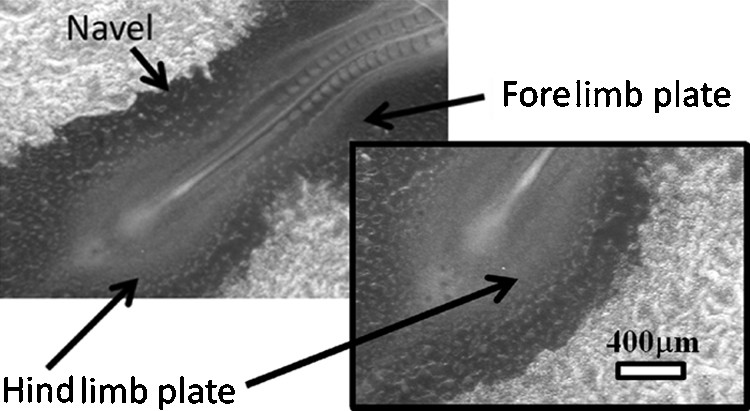
Chicken embryo, 16 somites (HH 12), Mag. 2.5X, Full scale 4.8 mm. In the yolk-sac, small pools of islands form an apparently “random” distribution of capillary precursors. Actually, their distribution follows the paths of the earlier vortex flow (see box magnifying the hindlimb plate). Note that the presumptive navel area shows a strong Left-Right constriction, segregating the rostral and caudal halves of the animal. The limb buds bulges start to form in the areas of the previous tissue winding.
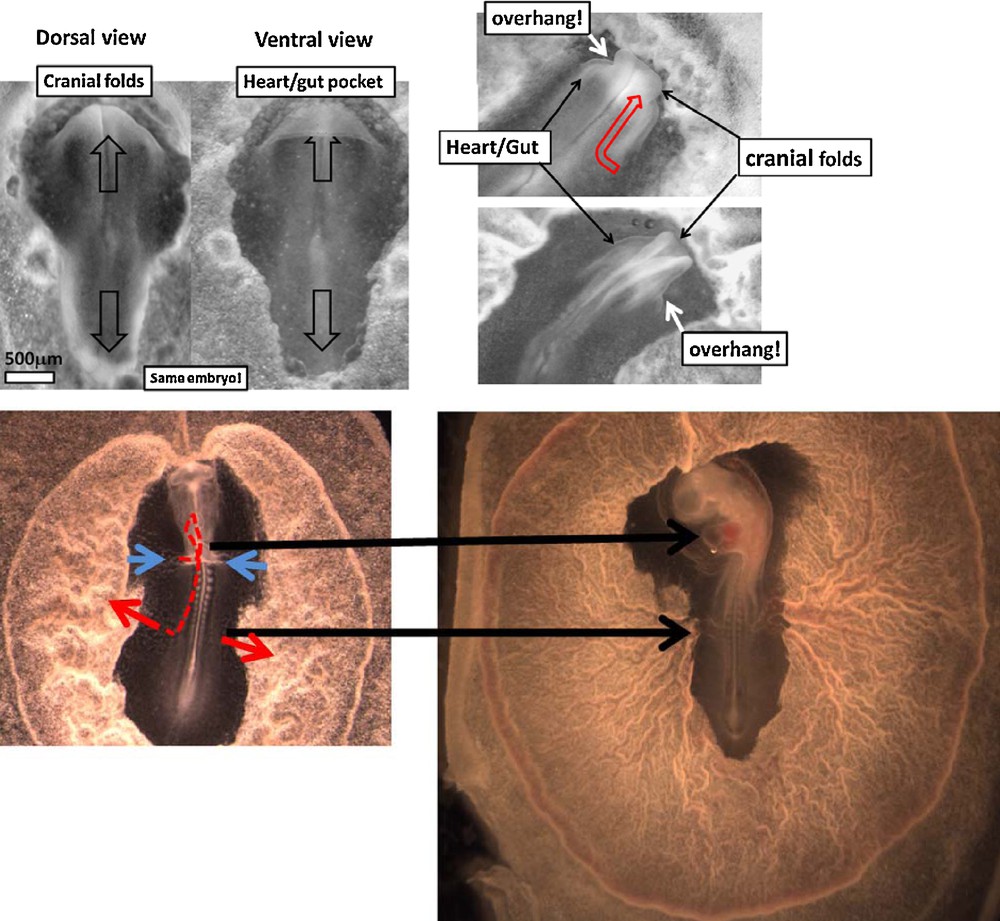
(A). Left: Dorsal and ventral views of an embryo at the moment of closure of the neural folds (HH 5), Mag. 4X. The tissue along the median axis passes over the gut/cardiac arch, generating a true fold edge, which marks the locus of the cardiac presumptive veins. Right: Two stages of rostral development showing how the continuation of the extension of the embryo in the cranial area (red arrow) leads to an overhang of the head above the gastrula plane, and formation of the heart fold (by contraction of the gut fold). After passing over the gastrula plane the head moves forwards, while the heart fold moves posteriorly (see Movies 7–10). (B). Left. At the stage of 9 somite pairs (HH 9–10), the first circulation loop is well-established. The bidirectional movement of the tissue in the hyperbolic point region has opened a path for the extraembryonic blood vessels to the embryo through the flanks (red arrows). The blue arrows point to the venous tract. Right. Chicken embryo HH 10. Vascular maturation forms progressively the classical image of the embryo resting on the yolk-sac, with vitelline arteries and veins connected to the navel. (see also Supplementary Fig. 7).
The heart itself (Fig. 8(A), Movies 6–8, see also [14]) forms from a fold located inside the gut pocket which appears in the distal parts of the embryo proper perpendicular to its median axis, i.e. downstream of the hyperbolic flow directed cranially. As the embryo extends forward, the median dorsal folds pass over the embryo plane (Fig. 8A)). This movement generates a sharp ventral folded edge of the underlying endoderm, which wraps the internal visceral mesoderm like dough. This is the first primordium of a heart, topologically complete. Next this edge constricts, as the head passes above it to become a heart [14]. Due to the fold topology, the edge forming the heart connections to the presumptive yolk stalk moves in the caudal direction while the head fold moves in the cranial direction. As the heart constricts, the vitelline veins form by wrapping of the mesoderm and its haemangioblasts within (Fig. 8(B) and Supp. Fig. 6, see also the movies of complete heart formation). Hence they are spontaneously, “automatically”, connected with the yolk-sac and heart, forming the first circulation loop.
To conclude this part, the films and pictures demonstrate that the establishment of the vascular pattern of amniotes is a consequence of the hyperbolic flow, which contributes to prepatterning of the vascular bed of the avian “umbilical” region along the streamlines of the flow and generates the tubular structures of heart and major blood vessels, by viscoelastic folding of underlying endoderm and mesoderm, in directions perpendicular to the flow.
5 Conclusion
We have shown that the material property of the embryonic tissue is that of a viscoelastic material, viscous in the long term (for time scales >5 s). We have observed tissue movements which are qualified as fluid speeds. We have followed the early stages of chicken development, from early gastrulation stage, to the stages when the body plan is recognizable (approx. 36 h of development). We were able to extract the vector fields of the tissue flow by particle tracking techniques with great accuracy, in part because the chicken embryo is quite flat, as compared to other animal models, and because our simple optical technique generates very crisp images by grazing illumination. We have demonstrated that vertebrate morphogenesis is a matter of a coherent hyperbolic flow around a stagnation point in the area of the future yolk sac stalk. The Supp. Fig. 8 summarizes the tissue flow of the ectoderm. The mesoderm and ectoderm flow on each other with relative speeds correlated by the principle of action and reaction. Embryo development is found to be bidirectional from the presumptive “umbilical” region. The tissue winds with opposite chiralities in four domains which colocalize with the limb fields.
The rostral extension of the embryo generates the heart by a process of endoderm and mesoderm folding and contraction, when ectoderm along the midline of the embryo is pulled straightforward by the flow, and starts to overhang above the blastula plane. In the yolk stalk area (navel analog), a funnel forms towards the yolk sac which follows the flow streamlines. The latter prepare the path for vascular development. Until the 15 to 20 somites stage, the somites are found upstream the yolk sac stalk area, this is to say that they are systematically advected away from the yolk sac stalk in the rostral direction, contrary to common knowledge. Actually the wave of somites formation occurs on a tissue which moves bidirectionnaly, hence all somites appearing above the stagnation point are systematically advected away rostrally.
The global animal pattern shows that there exists an organization satisfying the principle of Curie, by which the symmetry of the effect is to be found in the symmetry of the cause [8]. This proves, or at least suggests, that the positioning of chicken limbs are an effect of the hyperbolic topology which splits the vector field of development of the early blastula into four domains. The final pattern inherits this topology regardless of the actual force which drags the tissue, since at low fluid velocities, it is the initial symmetry-breaking which induces the topology, not the specific force field (no spontaneous symmetry breaking). The actual force driving the movement is likely to be cell–cell traction, since, on the one hand, folded ectoderm simply contracts like a purse along the edge [14]; on the other hand, the stretch upon the notochord is related to the mere change in boundary conditions along the median furrow, and to the continuous flow of mesoderm underneath; it is not clear whether any genetically induced discontinuity is truly necessary to explain the change in movements, which might be due to actual changes of the physics of the problem, as the cell layers move within finite boundaries. Also the Movie 4 shows a clear contraction in the area of the yolk sac stalk, as the neural crest closes.
It must be insisted that vortex pairs, as observed here, have their own emergent properties [3], such as self-organized flow in opposite directions. It is therefore tempting to suggest that hydrodynamic laws (conservation law, Stokes flow, boundary conditions) contribute to self-organization of the entire vertebrate bauplan, especially of the limb windings, and that the concept of information position encapsulated in chemical gradients misses the true nature of embryogenesis.
1 The yolk stalk of birds is located in approximately the same region as the umbilical chord of mammals, although they do not derive from exactly the same tissues. It is likely that the dynamics of the process is strong enough to rake the tissues towards the stagnation point, even with different initial conditions.
2 We observe that the endoderm is much thinner than the ectoderm, and quite transparent.
3 Except the first 3 of them.