1 Introduction
Potato (Solanum tuberosum L.) is one of the four staple foods in the world and the average consumption per capita is gradually elevated since it is well known for a dietary health food. Moreover, it takes a relatively short period (about three months) for harvesting in field with a high production rate per unit acre, i.e. an annual production exceeding 300 million tons (http://faostat.fao.org). However, potato is known to be vulnerable to several environmental stresses, such as drought and high temperatures. Even short periods of stress with high temperature can result in the serious damage and cause a significant reduction in tuber yield [1]. Therefore, in view of global warming, developing a potato line tolerant to such environmental stresses became one of the major tasks for potato breeders. Recently, several attempts have been made at creating potato crops possessing enhanced tolerance to such environmental stresses by overexpressing genes involved in the osmotic adjustment [2,3], transcription factors for the drought-signaling pathway [4] involved in the phytohormone's metabolic pathway [5], and genes responding to oxidative stresses, such as strong light, UV, and H2O2 [6,7].
In plants, carotenoids act as a strong antioxidant, which prevents damage from various environmental stresses, including strong light, high temperature, UV, and drought. In addition, carotenoids are also a major source of vitamin A to animals and humans who are unable to synthesize by themselves [8]. Therefore, many efforts have been focused on the elevation of carotenoid contents in several important crops, including rice, maize, and potato. Various carotenogenesis-related genes have been introduced into potato for the elevation of carotenoid content in its tuber by using overexpression [9–11], co-transformation with more than two genes [12], anti-sense approach [13–15], and RNAi technology [16]. In particular, Diretto et al. [12] reported that β-carotene has been elevated up to 3600-fold in tuber compared to control by co-transformation of microorganism's CrtB, CrtI and CrtY gene encoding phytoene synthase, phytoene desaturase, and lycopene β-cyclase, respectively. Most of the previous works for the elevation of the carotenoid content have been focused on the accumulation in sink tissue, tuber for the improvement of nutritional quality, but much less attention has been given to the leaf tissues for the development of enhanced tolerance against various environmental stresses.
In 2002, Davison et al. [17] reported that the overexpression of β-carotene hydroxylae enhanced the stress tolerance in Arabidopsis. They proposed that the enhanced stress tolerance was due to the increased size of the xanthophyll cycle pool, the reversible interconversion of two carotenoids, violaxanthin and zeaxanthin, which play a key photoprotective role in plants [18]. The transgenic plants were more tolerant to high light and high temperature, exhibiting reduced necrosis and lipid peroxidation. It was also reported that the overexpression of bacterial β-carotene hydroxylase gene (crtZ) involved in the conversion step of β-carotene and β-cryptoxanthin to zeaxanthin significantly enhanced UV tolerance in tobacco by elevating the zeaxanthin content [19]. Based on this observation, it was suggested that the enhanced functional xanthophylls cycle pool by the overexpression of crtz gene played a major role for the increased tolerance to UV stress and damage. In transgenic sweet potato calli, the silencing of the β-carotene hydroxylase (CHY-β) gene also showed a significantly enhanced tolerance to salt meditative oxidative stress along with higher levels of β-carotene [20]. Moreover, the silencing of another gene, lycopene ɛ-cyclase (LCY-ɛ) that is involved in the first step of the α-branch synthesis pathway of carotenoids from lycopene also resulted in the 21-fold elevated content of β-carotene in sweet potato calli and enhanced a significant tolerance to salt mediated oxidative stresses [21]. However, it is still largely unknown about the mechanism involved in the enhanced tolerance activity by the elevation of the carotenoid content.
Previously, we were able to isolate the Or gene from sweet potato and create a transgenic sweet potato calli exhibiting a 14-fold increased level of total carotenoid along with enhanced tolerance activity to salt stress through the overexpression of the Or gene [22]. Although the function of the Or gene is still largely unknown, it has been suggested that the Or gene is involved in the differentiation of proplastids or non-colored plastids into chromoplasts for carotenoid accumulation [23]. Furthermore, the microscopic examination proved that the high accumulation of carotenoid was due to the formation of chromoplasts containing carotenoid-sequestering structures [24]. In addition, Li et al. [25] reported that the elevation of the carotenoid content was effective, even during the post-harvest storage of potato tuber. Based on these previous results, it has been proposed that the manipulation of the Or gene will provide a better strategy for the enhanced carotenoid content in a storage organ like tuber than the manipulation of a specific gene involved in the metabolic pathway of carotenoids.
In this study, we tried to introduce the IbOr gene isolated from sweet potato into potato to further examine the function of the IbOr gene in the accumulation of carotenoid in a heterologous organism and observe the tolerance activity against various environmental stresses.
2 Materials and methods
2.1 Plant materials and vector construction
Potato plant (S. tuberosum L. cv. Atlantic) was maintained in vitro by sub-culturing every four weeks at 23 °C under 16-h light (4000 Lux) and 8-h night conditions, as described by Goo et al. [26]. Microtubers were induced by culturing continuously in vitro without sub-culturing for longer than two months. In every transformation experiment, fresh plants were obtained from newly induced microtubers by culturing on solid MS media [27] containing 2% sucrose. A recombinant T-DNA vector was constructed by placing the IbOr gene isolated from sweet potato (GenBank accession no. HQ828087) under the direction of SWAP2 promoter, a strong oxidative stress-inducible peroxidase promoter [28] and nos terminator. For the selection of transgenic lines, a bar gene encoding the phosphinothricin acetyl transferase was placed between the CaMV35S promoter and the nos terminator.
2.2 Transformation
The explants were prepared from the leaves of fresh plants of microtubers induced from an in vitro maintained plant prior to transformation and co-cultured with Agrobacterium. tumefaciens GV3101 bearing a recombinant vector as previously described [29,30]. Briefly, after co-cultivation with A. Tumefaciens carrying recombinant vector, they transferred on a regeneration medium (MS salts, sucrose 30 g/L, NAA 0.01 mg/L, zeatin 2.0 mg/L, GA3 0.1 mg/L, carbenicillin 500 mg/L) and maintained until the regeneration of shoots. Regenerated shoots were then placed on the selection media containing MS salts plus sucrose 30 g/L, phosphionthricin (PPT) 0.5 mg/L, carbenicillin 250 mg/L until rooting. When it is necessary, a higher concentration of PPT (up to 2.0 mg/L) was tried to enhance the fidelity of the transformation before molecular characterization.
2.3 Nucleic acid extraction, polymerase chain reaction (PCR) and reverse transcriptase (RT)-PCR
Genomic DNA from regenerated plants was isolated with GeneAll®Exgene™ Plant SV kit (Seoul, Korea) as described in the handbook provided. PCR was carried out with purified genomic DNA and primer set for the IbOr gene indicated in Table 1. A primer set for bar gene was designed as follow: 250F, 5′-CGGTCTGCACCATCGTCAACC-3′ and 251R, 5′-GTCCAGCTGCCAGAAACCCAC-3′. To purify total RNA from the plant, leaves or tubers were macerated in liquid N2, and Trizol reagent (Invitrogen, California, USA) was used to extract RNA, as described in the manufacturer's protocol, which was further purified with RNeasy Plant Mini kit (Qiagen, Germany). cDNA was synthesized with a kit (Toyobo, ReverTra Ace®-αE-, Osaka, Japan). PCR and RT-PCR products were fractionated on 1.5% agarose gel.
Summary of transgenic plant.
Vector | No. of explants (A) | No. of regenerated shoots | No. of resistant plant in PPT (1.0 mg/L) | No. of transgenic plants (PCR) | No. of transgenic plants (RT-PCR) (B) | Transformation efficiency (B/A × 100) |
SWAP2:IbOr:pPZP-Bar | 426 | 210 | 39 | 23 | 23 | 5.4% |
2.4 Quantitative real time-PCR (qRT-PCR)
Purified total RNA from either leaf or microtuber of potato plants maintained in vitro was used to qRT-PCR for the detection of transcript levels. The reaction was carried out by using an Mx300P qPCR system (Agilent Tech. CA, USA) as described in the instruction manual provided. Briefly, 200 ng of cDNA template were mixed with 10 pmol of each specific primer set and iTaq™ Universal SYBR®Green Supermix (Bio Rad, CA, USA) and adjusted to a final reaction volume of 20 μL. Then, it was initially denatured at 95 °C for 10 min and followed by 30 cycles of 30 s at 95 °C, 30 s at 58 °C, 1.0 min at 72 °C. At the end of the 30th cycle, it was extended at 72 °C for 10 min. Actin gene was used as an internal control for normalization. The relative expression levels of carotenogenesis-related genes were calculated using the 2−ΔCT method, as described in the instruction manual provided by Agilent Tech. (CA, USA). All data are the means of three replicates from at least three individual experiments.
2.5 Carotenoid analyses
To analyze the composition of carotenoids, HPLC analyses were carried out as previously described [31–33]. Briefly, 250 mg of the lyophilized samples were homogenized in a pre-chilled mortar and a pestle with 15 mL of acetone (0.01% butylated hydroxytoluene, BHT), sea sand, Na2SO4, and NaHCO3. The solution was transferred to a 15-mL conical tube and sonicated three times for 10 min. The extract was centrifuged at 7000 rpm, 4 °C for 10 min (Eppendorf 5430R, Germany), and 5 mL of the supernatant were dried under a flow of N2 gas and dissolved in 500 μL of a CH2Cl2:acetone mixture (1:1, v/v). This sample solution was filtered through a 0.45-m membrane filter (Whatman, PTFE, 13 mm) prior to HPLC analysis.
The Agilent 1100 HPLC system (Hewlett-Packard, Waldbronn, Germany) consisted of a temperature controlled autosampler, column oven and binary pump. A 20-μL volume of standard or sample solutions was directly injected in a YMC C30 carotenoid column (3 μm, 4.6 × 250 mm, Japan) with solvent A [methanol-tert-butylmethyl ether:water (81:15:4, v/v)] and solvent B [methanol-tert-butylmethyl ether:water (6:90:4, v/v)], using a step gradient elution of 100% A for the first 10 min, then 100% A to 100% B for the next 35 min. A conditioning phase (45–50 min) was used to return the column to its initial state. The flow rate was 0.7 mL/min and the column temperature was set at 25 °C. The eluent was detected with a UV–visible detector at 450 nm. The Chemstation software (Hewlett-Packard, Avondale, CA, USA) was used to monitor the HPLC-DAD system.
Carotenoids were quantified using an external calibration method. One milligram of each standard was dissolved in 10 mL of dichloromethane, containing 0.01% BHT. Working calibration solutions (50, 20, 10, 5.0, 2.5, 1.0, 0.50, 0.25, 0.10 and 0.025 μg/mL) were prepared by diluting the stock solution of the external standard. Standards of violaxanthin, lutein, all-trans-β-carotene and 9Z-β-carotene were purchased from CaroteNature GmbH, Lupsingen, Switzerland. In these chromatographic conditions, standard carotenoids gave peaks at the following tR (min): 13.2 for violaxanthin, 24.1 for lutein, 39.6 for all-trans-β-carotene and 40.9 for 9Z-β-carotene. Methanol, water and tert-butylmethyl ether used in the HPLC system were all of HPLC grade and the other chemicals were extra grade.
2.6 Stress treatment and analyses of tolerance activity
Transgenic plants and non-transgenic control plants were grown for four weeks on MS solid media containing 3.0% sucrose and microtubers were induced as previously described. For the treatment of NaCl- or MV-mediated oxidative stress, the leaf and sliced microtuber (200 mg) was treated with 300 mM of NaCl for 24 h and 3.0 μM of methyl viologen (MV) dissolved in 0.4% sorbitol for 24 and 48 h, as previously described [7,21]. To measure the tolerance to MV-mediated oxidative stresses, NaCl treated tissues were incubated in a solution containing 1 mg/mL of 3,3-diaminobenzidine (DAB)-HCl (pH 3.8) for 5 h at 25 °C under continuous light, as described by Chadwick et al. [34] and Kim et al. [22]. The percentage of ion leakage by MV treatment was assessed using an electrical conductivity meter (Thermo Scientific Orion 3Star conductivity Benchtop, Maltham, MA, USA) at a specified time point, 24 and 48 h. At the end of the specified time period, the samples were autoclaved for 15 min at 121 °C to release all of the solutes and the conductivity were re-measured for the calculation of relative ion leakage based on this value at each time point. The radical-scavenging activity of 1,1-diphenyl 2-picrylhyorazyl (DPPH) was assessed as previously described, with slight modification [22,35]. Briefly, 200 mg of leaf or sliced microtuber tissues were ground MeOH and centrifuged at 15,000 g for 5 min. An aliquot of supernatant (100 μL) was mixed with 750 μL 0.5 mM DPPH in MeOH and shaken vigorously, left in the dark at room temperature for 30 min. The relative DPPH radical-scavenging activity was assessed by measuring the absorbance of the reactant at 517 nm with a spectrophotometer (Shimadzu, Kyoto, Japan). A MeOH solution was used as a blank for the measurement of absorbance.
2.7 Statistical analysis
All of the measurements for carotenoid content, tolerance activity for NaCl and MV-mediated oxidative stresses and DPPH radical-scavenging activity were repeated three times. The results are shown as the mean values with the standard deviation (SD).
3 Results
3.1 Transformation efficiency and molecular characterization
The constructed recombinant plasmid (SWAP:IbOr:pPZP-Bar) was initially transformed into A. Tumefaciens, GV3101 and then into potato by co-culturing with explants prepared from fresh shoot of newly induced microtubers. In total, 426 explants were co-cultured to regenerate shoots and selected on the media containing 1.0 mg/L of PPT through several individual experiments. Out of 39 plants that survived on the selection media containing PPT, 23 individual plants confirmed that the recombinant T-DNA construct was successfully inserted into their genome by using PCR and RT-PCR (Table 1). The frequency of transgenic plants was 5.4% and as shown in Fig. 1, in all plants, both IbOr and bar gene fragments were detected by PCR and their transcripts were also detected by RT-PCR, but not the negative control plant, non-transgenic plant, Atlantic cultivar, suggesting that the IbOr gene is successfully integrated into the genome of potato and actively transcribed under the stress inducible promoter, SWAP2.
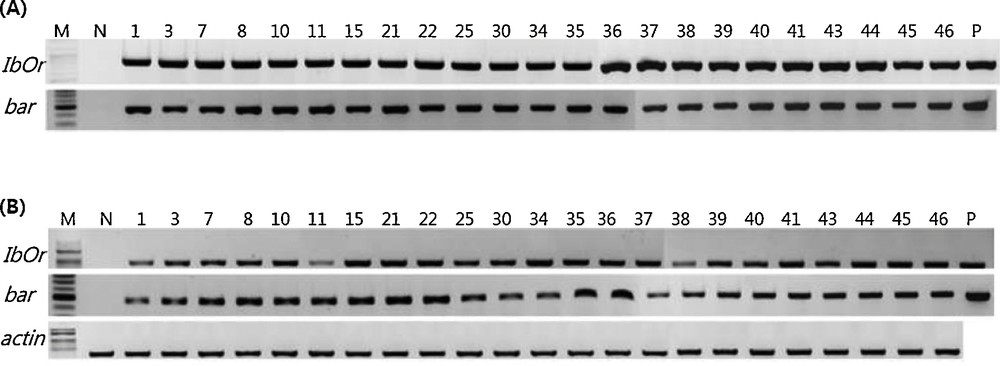
Results of PCR and RT-PCR for the confirmation of transgenic potato plants bearing the IbOr gene. A. Genomic DNA was purified from candidate transgenic potato plants and PCR was carried out with primer sets for IbOr or bar gene as described in Materials and methods. B. Total RNA was purified from candidate transgenic potato plants and RT-PCR was carried out with primer sets for IbOr, bar and actin, as described in Materials and methods. PCR and RT-PCR products were fractionated on 1.5% agarose gel. M: size marker; N: negative control, non-transgenic parental line, Atlantic cultivar; P: positive control.
3.2 Enhanced level of carotenoids content in transgenic potato overexpressing IbOr
To select transgenic plants accumulating higher content of carotenoids, we then performed HPLC analyses with harvested microtubers from transgenic lines confirmed by molecular analyses. As summarized in Table 2, three individual transgenic lines, SOR3, SOR45 and SOR46 were selected, since they accumulated higher contents of carotenoids than that of the non-transgenic control cultivar. In detail, SOR46 line accumulated the highest content of total carotenoid (about 38.1 μg g−1 DW), 2.7-fold increased level compared to that of non-transgenic control cultivar. When calibrated with each peak of external standard carotenoid, violaxanthin, lutein, and β-carotene were enhanced up to 2.4- to 3.2-fold, whereas 9Z-β-carotene, 13Z-β-carotene, α-carotene, zeaxanthin, and cryptoxanthin were not detected at all, even in the non-transgenic control. The other two individual transgenic lines, SOR3 and SOR45 also exhibited 1.4- to 2.1-fold elevated total carotenoid content, and violaxanthin, lutein and β-carotene were increased at similar level but the other five carotenoids measured in this study were not detected at all. Our results indicate that the overexpression of the IbOr gene does not influence the accumulation pattern of pre-existing carotenoids in the non-transgenic control plant, but only enhance the levels of carotenoid that are already present in the non-transgenic control, although the expression of the IbOr gene was directed under the stress-inducible promoter.
Carotenoid content and composition in micro-tuber of transgenic potato overexpressing IbOr gene measured by HPLC analyses.
Line | Violaxanthin | Lutein | β-Carotene | 9Z-β-Carotene 13Z-β-Carotene |
α-Carotene, zeaxanthin, cryptoxanthin |
Others | Total |
Tuber | |||||||
Atlantic | 7.5 ± 1.1 | 3.2 ± 0.3 | 1.2 ± 0.0 | ND | ND | 2.1 ± 0.2 | 13.9 ± 1.6 |
SOR3 | 10.5 ± 1.6 | 5.6 ± 0.9 | 1.7 ± 0.1** | ND | ND | 2.5 ± 0.2 | 20.0 ± 2.7* |
SOR45 | 16.8 ± 0.2** | 9.2 ± 0.0** | 2.4 ± 0.0** | ND | ND | 1.4 ± 0.0* | 29.7 ± 0.2** |
SOR46 | 23.6 ± 0.6** | 10.0 ± 0.2** | 2.7 ± 0.1** | ND | ND | 1.8 ± 0.3 | 38.1 ± 0.9** |
* P <0.05.
** P < 0.01
3.3 Effect of IbOr overexpression on the transcript levels of carotenogenesis-related genes in transgenic potato
We then examined the transcript levels of 11 genes that are involved in the complicated carotenoid biosynthetic pathway by using qRT-PCR from three individual transgenic lines, SOR3, SOR45, and SOR46. Specific primer sets for each gene's amplification were designed from the information as described in Table 3 and the results obtained by qRT-PCR were normalized against internal control, actin's transcript level and summarized as shown in Fig. 2. The IbOr gene was actively transcribed in all transgenic lines, while no transcript was detected in the non-transgenic control, Atlantic. In general, the transcript levels of 11 genes were higher in tubers than in leaves. In tuber, the expression levels for most of genes were elevated compared to the non-transgenic control, Atlantic, while most of genes were maintained at similar levels in leaves, except the Zep gene. In detail, the transcript level of Zep was most dramatically increased in both leaf and tuber tissues (up to 1.6-fold in leaves and 5.6-fold in tuber). The transcript levels of Psy2, PDS, ZDS, Crtlso, Lcy-e, and Zep in SOR46, which accumulated the highest level of carotenoids, were elevated up to 6.0, 4,6, 5.6, 8.0, 6.0 and 14.8-fold, respectively.
Primers used for qRT-PCR analyses of carotenogenesis-related genes in transgenic potato overexpressing the IbOr gene.
Gene name | Accession number | Direction | Primer sequences 5′–3′ | Amplicon Tm (°C) |
Amplicon length (bp) |
Or | HQ828087 | For | GGAAGAGGTTCGTAGGCTGA | 53.2 | 106 |
Rev | CAGGTTAGGGTTCTGGGATTT | 53.5 | |||
Actin | J01298.1 | For | GGCTGGATTTGCTGGTGATG | 57.4 | 140 |
Rev | CCGCCTGAATAGCAACATAC | 52.1 | |||
Psy1 | TC122598 | For | CGGTCTGCTATTGTTGCTACTCC | 56.7 | 141 |
Rev | CAGGAACAGGTATGTCTGGCTTC | 56.4 | |||
Psy2 | L23424 | For | AGCTTTAGATAGGTGGGAGGCA | 55.8 | 162 |
Rev | CAAGTCCATACGCATTCCTTCAA | 57.7 | |||
PDS | AY484445 | For | AGAGACTTTGCATGCCGATTGT | 57.4 | 151 |
Rev | AAAGCATCGCCCTCAACTGT | 55.9 | |||
ZDS | TC114158 | For | TTGCCATGTCAAAGGCCA | 55.3 | 141 |
Rev | ACAGGCACTCCGACCAATTT | 55.6 | |||
Crtlso | TC117194 | For | TTGGCAGCAGTAGGACGTAAAC | 55.8 | 151 |
Rev | TCCCTTCCTTTTCATGTGGAA | 55.6 | |||
Lcy-e | AF321537 | For | GCCAAAATGGATGTGGCAG | 55.9 | 151 |
Rev | CAATGTTGCACCAGTAGGATCAG | 55.9 | |||
Lut1 | TC117729 | For | CGTTCTCCGCCCAAAAAAC | 56.7 | 140 |
Rev | TTGGCCTAAAGTAAGTGACCTGG | 56.2 | |||
Lcy-b | X86452 | For | AATGGGTGGTCCACTTCCAGTA | 56.8 | 76 |
Rev | GGATGGATGAACCATGCCAG | 57.1 | |||
Chy1 | TC36005 | For | CTTGGCCCAAAACCCACTT | 56 | 152 |
Rev | CCTCAAATTGAGGTTTCAGCTTCT | 56.8 | |||
Chy2 | TC32024 | For | TTTTGCTGTCTCGAAGAAAGCC | 57.3 | 148 |
Rev | AGCCAACAGGCAGCTAAACTCT | 56.1 | |||
ZEP | EST724320 (K278242) |
For | TCATGAATGCTGGCTGCATC | 56.6 | 151 |
Rev | TGCTGCAAAGTCATGCGG | 56.2 |

Expression profile of various genes involved in the carotenoid biosynthetic pathway in transgenic potato overexpressing the IbOr gene measured by using qRT-PCR. Values are the means ± SD of three replicates from at least three individual microtubers or leaves. Atlantic is the parental recipient potato cultivar; the non-transgenic control and SOR series are transgenic potato lines. Act, actin; Or, IbOr; Psy, phytoene synthase; PDS, phytoene desaturase; ZDS, ζ-carotene desaturase; Crtlso, carotene isomerase; Lcy-e, lycopene ɛ-cyclase; Lut1, α-carotene hydroxylase; Lcy-b, lycopene β-cyclase; Chy, β-carotene hydroxylase; Zep, Zeaxanthin epoxidase.
3.4 Enhanced antioxidant activity and salt tolerance in IbOr transgenic potato plants
To evaluate the tolerance to antioxidants, the three individual transgenic lines (SOR3, SOR 45 and SOR 46) and non-transgenic control were grown in vitro for four weeks and microtubers were induced. For the measurement of tolerance to oxidative stress induced by salt, leaves and sliced microtuber discs (about 1.0 mm in thickness) were placed on MS solid media containing 300 mM NaCl for 24 h. When the cellular H2O2 content from NaCl-treated tissues was measured by calculating the oxidized DAB content after reaction in the DAB solution, both leaves and tuber tissues of three transgenic lines exhibited less amounts of oxidized DAB compared to those of the non-transgenic cultivar (Fig. 3A). In particular, in microtubers of the SOR46 line, the oxidized DAB amount was reduced up to 50%. We also measured DPPH radical-scavenging activity to further examine the enhanced antioxidant activity. All three transgenic lines showed enhanced activity in leaf as well as microtuber compared to the non-transgenic control, Atlantic. In detail, the leaf tissue of SOR46 line exhibited 2.4-fold enhanced activity while 1.3-fold enhanced activity was examined in microtubers (Fig. 3B). To measure the tolerance activity to MV-mediated oxidative stress, sliced microtuber and leaf discs were prepared from three transgenic lines and non-transgenic control and then treated with 3.0 μM MV in 0.4% sorbitol solution. The relative ion leakage was measured after treatment with MV, in which a typical ROS-generating redox-active compound is present. Compared to non-transgenic control plants, three transgenic lines also showed a lesser level of ion leakage in leaf as well as microtuber discs upon treatment with MV, indicating that the membranes in transgenic lines were less damaged that those of control plants. As expected by the elevated tolerance activity upon treatment with NaCl-mediated oxidative treatment, SOR46 line showed the lowest level of ion leakage in both leaf and microtuber discs at 24 and 48 h after MV treatment (Fig. 3C).

Tolerance activity against salt-mediated oxidative stress (A), MV-mediated oxidative stress (C and D) and DPPH radical-scavenging activity on non-transgenic (Atlantic) and transgenic potato (SOR series) overexpressing the IbOr gene. Leaf or tuber tissues were treated with NaCl (300 mM) for 24 h in the dark and their oxidation was measured by adding a DAB solution. The relative DPPH radical-scavenging activity was measured from a methanol extract of leaf or tuber tissues by adding a DPPH solution. MV (3.0 μM) in 0.4% sorbitol was treated on leaf or tuber tissues for 24 and 48 h in the dark and ion leakage was measured with EC meter. Values are the means ± SD of three replicates from at least three individual microtubers or leaves. Atlantic is the parental recipient potato cultivar; the non-transgenic control and SOR series are transgenic lines.
4 Discussion
In this report, we were successfully able to create several transgenic potato plants (S. Tuberosum L. Cv. Atlantic) overexpressing IbOr gene under the direction of a stress inducible promoter, SWAP2. Initially, 39 individual transgenic plants were confirmed by PCR and RT-PCR that the recombinant T-DNA was successfully introduced into the genome of potato and actively transcribed. The final transformation frequency for Atlantic cultivar was about 5.4% (Table 1) and it was less than that of Jowon cultivar (12.5%) previously reported [26], indicating that the transformation frequency of potato is dependent on genotypes.
HPLC analyses showed that three individual transgenic lines SOR3, SOR45, and SOR46 accumulated elevated contents of carotenoid compared to non-transgenic controls. Although the total carotenoid levels of these transgenic lines were elevated, the pattern of carotenoid composition was not changed. Non-transgenic control, Atlantic cultivar, parental recipient line for transformation accumulated violaxanthin, lutein, and β-carotene in its tuber but 9Z-β-carotene, 13Z-β-carotene, α-carotene, zeaxanthin, and β-cryptoxanthin were not detected at all. Only three carotenoids pre-existing in the non-transgenic cultivar, Atlantic, were elevated by the overexpression of IbOr gene in transgenic lines, but not the other five carotenoids examined in this study (Table 2). Our preliminary results were consistent with a previous report on the transgenic sweet potato calli overexpressing IbOr gene [22]. In sweet potato, α-carotene, zeaxanthin, lutein, β-carotene, and β-cryptoxanthin were already present in the non-transgenic controls and they were the only elevated carotenoids by overexpression of the IbOr gene. Interestingly enough, violaxanthin was not detected in non-transgenic sweet potato, whereas zeaxanthin was not present in non-transgenic potato control, Atlantic cultivar. These preliminary results were also consistent with previous observation in transgenic potato overexpressing the Or gene isolated from cauliflower by Lopez et al. [24]. They also found that the overexpression of the Or gene under the tuber-specific promoter, granule-bound starch synthase (GBSS) increased the levels of mainly violaxanthin, lutein and β-carotene, which were present in the non-transgenic potato tuber.
It was reported that the Or gene encodes a protein-like molecular chaperon involving the differentiation of chromoplast from non-colored plastids, and provides a metabolic sink to sequester carotenoids [23]. However, the detailed mechanisms are largely unknown. To further examine the function of the Or gene in the metabolism of carotenoids in heterologous organism, we looked into the transcription levels of carotenogenesis-related genes in transgenic plants. Most of genes including Psy, Pds, Zds, Crtlso Lcy-e and Zep accumulated higher levels of transcript in the tuber of transgenic plants compared to non-transgenic controls. Noticeably, the transcript level of Zep in tuber of SOR 46 line in which accumulated the highest content of total carotenoid was elevated up to 14.8-fold compared to non-transgenic controls. Interestingly, the content of violaxanthin that is converted from zeaxanthin by Zep was also elevated up to 3.2-fold. However, in leaves, most of genes examined in this study exhibited similar levels of transcripts compared to non-transgenic control (Fig. 2). Previous reports also found that the overexpression of the Or gene did not show a specific effect on inducing the expression of any particular gene in the transgenic potato tuber [24]. Instead, higher expression levels of several genes were observed as our results. In contrast, transgenic sweet potato calli accumulated increased levels of transcripts for most of the genes involved in carotenoid biosynthetic pathway as well as NCED involved in ABA biosynthesis and Pftf involved in chromoplast differentiation [22]. In particular, the Pftf gene showed a significant increase in transcript levels and strongly suggested that the increased level of carotenoid conferred by the overexpression of IbOr gene is due to an increased driving force in sink strength rather than to an increased expression of endogenous carotenoid biosynthetic pathway. Further research is still required to examine the function of Or in the differentiation of chromoplasts.
A metabolic engineering strategy to increase the content of violaxanthin and zeaxanthin for the enhancement of stress tolerance has already been suggested, since two carotenoids are involved in the xanthophyll cycle, which is a key photoprotective mechanism in plants [17,18]. The xanthophyll cycle is the reversible interconversion between zeaxanthin and violaxanthin, and it was suggested that it specifically protects thylakoid membrane lipids against photooxidation [18]. Therefore, we further examined the stress tolerance activity with transgenic potato lines accumulated higher content of violaxanthin along with lutein and β-carotene. In SOR46 exhibiting the highest content of carotenoids, both leaf and tuber tissues showed a significantly enhanced tolerance to NaCl and MV-mediated oxidative stress. In addition, the DPPH radical scavenging activity was also significantly increased by the overexpression of the IbOr gene (Fig. 3). Our results clearly indicated that the higher content of carotenoid including violaxanthin by IbOr gene in transgenic potato resulted in an enhanced antioxidant activity. Previous reports also suggested that increased carotenoid in transgenic sweet potato calli overexpressing IbOr gene correlated with higher DPPH radical-scavenging activity and tolerance activity against NaCl-mediated oxidative stress [22]. It was also reported that the increased carotenoid biosynthetic intermediates by genetic manipulation of genes involved in the carotenoid biosynthetic pathway combat cooperatively against reactive oxygen species to alleviate cellular damage upon various environmental stresses [20,36].
In conclusion, transgenic potato lines we developed here showed enhanced tolerance activity against salt- and MV-mediated oxidative stresses and DPPH radical-scavenging activity. Our preliminary results indicate that the manipulation of the IbOr gene is a possible strategy to develop a crop tolerant to salinity and other environmental stresses in addition to improve the nutritional quality by increasing the carotenoid content through the enhanced sink strength.
Disclosure of interest
The authors declare that they have no conflicts of interest concerning this article.
Acknowledgement
This work was supported by a grant from the Next-Generation BioGreen 21 Program (No. PJ008097), Rural Development Administration, Republic of Korea. We thank Mrs. Min-Kyung Lee for her excellent technical assistance.