1 Introduction
The concept of immobilizing reagents or probes onto polymer supports for use in chemistry and biology has received a great deal of attention. Since the activity of supported reagents depends on the accessibility of the active sites and is often limited by diffusion, considerable efforts are made to develop new polymer supports with improved capacity, accessibility and selectivity [1–4]. In this context, the technique of polymerisation in microemulsion, developed in the early 1980s, offers new opportunities [5]. Indeed, the polymerisation of oil component in oil-in-water microemulsions allows one to produce stable suspensions of ultrafine particles in the nanosize range (diameter smaller than 30nm), so called ‘microlatexes’ or ‘nanolatexes’, which exhibit very large specific areas of up to 400–500m2/g for nanoparticles in the 10–15-nm range [5–7]. Moreover, the well-defined structure of microemulsions affords a means to synthesize special polymer materials with high degrees of chemical functionalisation [8–15].
Metal-complexing polymers and supports have been proposed for a variety of purposes like separation-recovery of metal ions, catalysis, and sensors [16–21]. For such applications, well-defined highly functionalized polymer nanoparticles hold most promise in chemistry for the development of special nanomaterials and supported catalysts or reagents with improved activity as well as in life science for the development of nanocarriers, nanosensors and nanoprobes that are suitable for intracellular transport and measurements [10,11,22–24]. Cyclam 1 is a well-known ligand that exhibits high affinities for transition metal cations with a remarkable selectivity for cupric ions (stability constant K = 1027) [25], a selectivity that remains unchanged after anchoring to polymer backbones or resins [17,18]. We have recently described that cyclam-functionalized nanoparticles in the 12–20-nm diameter range can be prepared by a one-step microemulsion copolymerisation process with the polymerisable macrocycle 2 (Fig. 1 , pathway A) [11]. These nanoparticles exhibit a very high selectivity for cupric ions. In such particles resulting from copolymerisation, the cyclam residues are distributed between the core and the surface: the ligand accessibility depends on the polymerisation conditions and is closely related to the size [11]. The smaller the particles, the higher the surface-to-volume ratio, the higher the ligand accessibility [11].
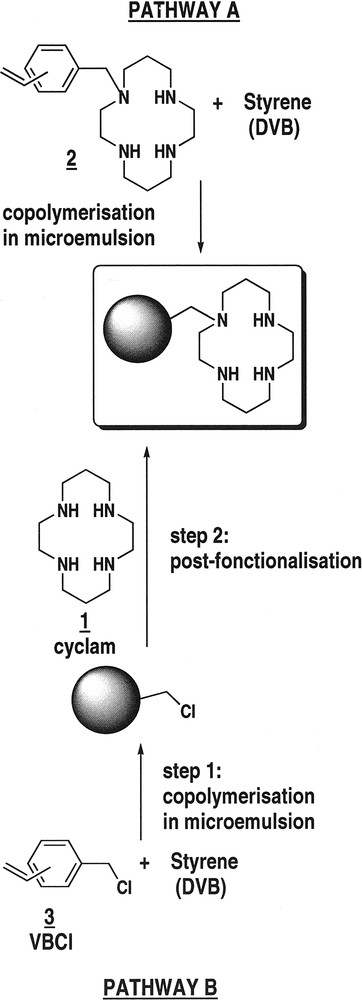
Synthetic pathways to cyclam-functionalised nanoparticles.
Ligand-functionalized nanoparticles may alternatively be obtained by post-grafting. Post-functionalisation of reactive nanoparticles could be a versatile method for binding various functional residues thus giving access to nanoparticles with adjustable functionalities and controlled size from a same microemulsion-polymerisation recipe. Although post-functionalisation of classical latexes and polymer gels has been widely used and is well documented [3], there are only few examples of such chemical modifications on nanoparticles prepared by microemulsion polymerisation [9,26]. For example, nucleophilic substitutions on polychloromethylstyrene microspheres or cross-linked resins have been found successful to introduce various chemical functions via reaction of nucleophilic anions or amines [18,27–30].
In this paper, we wish to report the synthesis of cyclam-functionalized nanoparticles by a two-step procedure: (1) microemulsion copolymerisation of vinylbenzylchloride 3 which produces reactive nanoparticles and (2) post-functionalisation with cyclam (Fig. 1, pathway B). For such a post-functionalisation process, the ligand is expected to be preferentially grafted at the surface of the nanoparticles. Then, we compare their metal binding capacity and their ligand accessibility with nanoparticles obtained by a one-step copolymerisation process.
2 Results and discussion
2.1 Preparation of reactive nanoparticles by copolymerisation of VBC 3 in microemulsion (pathway B, step 1)
Chlorobenzyl-functionalized nanoparticles are readily obtained by copolymerisation of vinylbenzylchloride 3 (15% mol) in ternary oil-in-water microemulsions (Fig. 1, pathway B, step 1). Microemulsions of mixtures of VBC 3 and styrene, with or without a cross-linking agent divinylbenzene (DVB), are prepared using a cationic surfactant DTAB (dodecyltrimethylammonium bromide) [10,11] and polymerized at room temperature using either an oil-soluble radical photo-initiator (DMPA: 2,2-dimethoxy-2-phenylacetophenone) or a water-soluble redox system (ammonium persulphate/tetramethyldiamino methane) [8–11]. In every case, polymerisation readily takes place reaching 100% conversion of all monomers within a few hours.
Whatever the experimental conditions, stable translucent aqueous suspensions of nanoparticles are obtained: the average diameters, determined by Quasi-Elastic Light Scattering (QELS), range from 15 to 23 nm with very narrow size distributions (Table 1). The smallest particles are obtained using the oil soluble initiator DMPA with no significant effect of the presence of cross-linking agent (exp. P1 and P2). It is worth noticing that repeated experiments lead to similar results (exp. P2.1 and P2.2). The particle size is quite similar to those obtained by polymerisation of styrene alone under the same experimental conditions (exp. P0 and P2), thus indicating that vinylbenzylchloride 3 is randomly distributed in the oil mesophase and does not contribute to the droplet surface coverage according to the previously proposed geometrical models [6,31]. Slightly larger particles, with an average diameter of 23 nm, are obtained when the water-soluble persulphate initiating system is used (exp. P3). In this case, the packing of the surfactant molecules in the interfacial area might be slightly modified by the formation of ion pairs with initiating anions and anionic oligomeric intermediates. Consecutive changes of the droplets size may account for the production of slightly larger particles.
Synthesis of the reactive nanoparticles by copolymerisation of VBC 3
Starting microemulsion(a) | Resulting nanoparticles | |||
Monomers load (%wt) | Initiation(b) | D(c) (nm) | Halogen content(d) (mmol g–1) | |
P1 | Styrene (3.2) | A | 16 | |
3 (0.75) | Cl: 0.75 | |||
%mol 3: 13.7 | Br: 0.39 | |||
mmol 3 g–1: 1.24 | Total: 1.14 | |||
Styrene (1.4) | Cl: 0.28 | |||
DVB (1.8) | Br: 0.43 | |||
P2.1 (e) | 3 (0.7) | A | 15 | Tot: 0.71 |
P2.2 (e) | %mol 3 : 14.4 | A | 16 | Cl: 0.38 |
mmol 3/g : 1.17 | Br: 0.41 | |||
Total: 0.79 | ||||
P3 | Styrene (1.4) | B | 23 | Cl: 1.07 |
DVB (1.4) | Br: N.D. | |||
3 (0.7) | ||||
%mol 3: 15.9 | ||||
mmol 3/g: 1.31 | ||||
P0 (f) | Styrene (1.7) | A | 14 | — |
DVB (2.2) |
(a) Microemulsions prepared in the presence of DTAB, DVB: divinylbenzene.
(b) Polymerisation reactions carried out at room temperature, initiating systems A = DMPA or B = persulphate/TMDAM.
(c) Average diameter deduced from QELS experiments (±2 nm); in every case the polydispersity index calculated by the cumulative method ranges from 0.02 to 0.1.
(d) Halogen content in the isolated and purified polymer deduced from elemental analysis (±0.03 mmol g–1).
(e) Identical experiments illustrating the reproducibility.
(f) Blank experiment: polymerisation without VBC 3.
The colloidal suspensions of nanoparticles are stable over very long periods of time (more than 1 year) and remain stable without particles aggregation when the surfactant concentration is reduced down to 1.5 wt% by dialysis.
The content of reactive groups is deduced from elemental analyses of the resulting isolated and purified polymer. The chlorine content as well as the presence of bromine in the polymer clearly indicates that two competitive side reactions, hydrolysis and exchange of chlorine for bromine, take place on chlorobenzyl groups (Table 2 and Fig. 2 ). The substitution of chlorine for bromine arises from the large amount of bromine anions introduced with the cationic surfactant: the overall Br/Cl molar ratio is about 10 in the reaction medium. Moreover, the local concentration of bromine counterions associated with the cationic surfactant at the interfaces is expected to favour the nucleophilic substitution on the starting monomer VBC 3 during the polymerisation as well as at the surface of the nanoparticles after polymerisation. Such a microenvironmental effect has already been proposed to account for the enhancement of Cl per Br substitution rates in micellar solutions of ammonium bromide surfactant [32]. If one considers the following post-functionalisation step, this substitution is profitable because it transforms benzylchloride groups into more reactive benzylbromide residues. The other side reaction is hydrolysis of the benzylhalide moities that produces non-reactive benzylalcohol residues and could reduce the ligand load in the final polymer. Similarly, hydrolysis might be favoured by the local concentration of hydroxide anions associated with the cationic surfactant in the interfacial region.
Metal-complexing nanoparticles: synthesis by post-functionalisation and binding properties
Post-functionalisation | Resulting nanoparticles | |||||
Starting suspension n equiv cyclam(a,b) | Yield(c) | Cyclam content(d) (mmol g–1) | D(e) (nm) | Cu-binding capacity(f) (mmol g–1) | ||
P2a | P2.1 | 2 | 35 % | 0.28 | 17 | 0.28(g,h) |
P2b | P2.1 | 0.5 | 25 % | 0.20 | 14 | 0.18(g) |
P2c | P2.2 | 4 | 34 % | 0.27 | 17 | N.D. |
P1a | P1 | 2 | 21 % | 0.24 | 16 | 0.24(h) |
P3a | P3 | 2 | 30 % | 0.23 | (i) | N.D. |
(a) Reaction carried out at room temperature for three days, starting suspension (see Table 1) and number equivalent of cyclam added per VBCl initially introduced.
(b) Number of equivalent of cyclam introduced calculated referring to the amount of VBC 3 introduced in the starting microemulsion.
(c) Substitution yield calculated from to the amount of halogen (Cl + Br) in the starting particles.
(d) Amount of cyclam deduced from nitrogen elemental analysis of the isolated and purified polymer (±0.03 mmol g–1).
(e) Average diameter from QELS experiments (±2 nm), polydispersity indexes are unchanged after functionalization.
(f) Binding capacity at the equilibrium in the presence of an excess of cupric ions.
(g) From copper elemental analysis of the isolated and purified polymer (±0.03 mmol g–1).
(h) From spectrophotometric measurements.
(i) Progressive aggregation was observed during QELS experiments.

Side reactions on starting monomer during polymerisation or at the surface of the particles.
As can be seen in Table 1, the extent of side reactions varies with the polymerisation conditions. Comparison of exp. P2 and P3 demonstrates that, for a given microemulsion recipe, both hydrolysis and Cl/Br substitution rates dramatically depend on the nature of the initiating system. When the persulphate redox system is used (exp. P3), the final chlorine content in the particles, about 1.1 mmol g–1, is close to the amount of VBCl 3 initially introduced. The substitution and hydrolysis rate does not exceed 15%. On the other hand, when the oil-soluble initiator DMPA is used (exp. P2), both Cl/Br substitution and hydrolysis readily take place. 35 to 40% of the chlorine atoms have been substituted by bromine. The overall halogen (Cl + Br) content in the particles is around 0.7 to 0.8 mmol g–1, indicating that about 35% of the benzylchloride residues have been hydrolysed. Electrostatic interactions may account for these results. When persulphate is used, the initiating species is a sulphate radical anion so that both the oligomeric intermediates and the final polymer are negatively charged. In this case, electrostatic repulsions with nucleophilic anions Br– and OH– inhibit side reactions. Such an inhibition has already been observed in the presence of anionic surfactants [9]. On the other hand, when DMPA is used, its decomposition produces neutral radicals: both the oligomeric intermediates and the resulting polymer are uncharged. The approach of the nucleophiles is not hindered by repulsive interactions. Moreover, ionic attractions with the cationic surfactant adsorbed in the interfacial region may increase the local concentration of nucleophilic anions and favour side reactions.
For a given initiator, DMPA, the comparison of exp. P1 and P2 indicates that the extent of hydrolysis is much smaller (less than 10%) in the absence of cross-linking agent than in the presence of cross-linking agent (about 35%). On the other hand, the rates of exchange of chlorine for bromine are quite comparable. The influence of the presence of DVB as a cross-linking agent on the hydrolysis rate may result either from changes in the kinetic of polymerisation or from differences in the swelling behaviour of the nanoparticles. A previously reported study of the suspension copolymerisation of VBCl 3 indicated that the benzyl chloride group on the monomer appeared to be more susceptible to hydrolysis than on the final polymer beads. Most hydrolysis was believed to occur in the early stages of the polymerisation [33]. In our experiments, polymerisation of VBCl might be slightly delayed in the presence of DVB thereby accounting for the observed increase of the hydrolysis rate. Further kinetic studies are under investigation in order to confirm this assumption.
On the other hand, it has been already reported that the polymer chains are highly compressed in nanoparticles resulting from microemulsion polymerisation in the absence of cross-linking agent [5]. Once the nanoparticle is formed it will tend to reject the water thereby minimizing hydrolysis. On the contrary, when prepared in the presence of DVB, the polymer matrix could have a sufficient structural rigidity to prevent collapse thereby limiting de-swelling. The highly cross-linked nanoparticles might thus retain a water swollen microgel-like nanostructure that favours hydrolysis. Such an influence of cross-linking on the microstructural features and swelling properties of polymer particles in water has already been reported [34,35].
2.2 Post-functionalisation with cyclam (pathway B, step 2)
Post-functionalisation is carried out under very mild conditions in the aqueous suspensions without pre-purification or separation of the nanoparticles [9]. The grafting of cyclam onto reactive chloro/bromobenzyl-functionalized nanoparticles is performed at room temperature by adding cyclam directly to the aqueous suspensions obtained by polymerisation in microemulsion. Unreacted cyclam is easily removed from the suspension by dialysis. Elemental analyses of the resulting isolated and purified nanoparticle demonstrate that the nucleophilic substitution occurs with a maximum content of 0.25–0.3 mmol of macrocyclic cyclam residues per gram of polymer (Table 2). The data reported in Table 2 show that maximum functionalisation yields are achieved in the presence of a slight excess of cyclam (2 equiv). Noteworthy, reactions performed in the presence of substoichiometric amounts of cyclam give acceptable functionalisation rates (0.2 mmol g–1). Under similar experimental conditions, the amount of grafted cyclam residues is quite comparable whatever the starting suspension.
For nanolatexes prepared using DMPA as an initiator, the particle size remains almost unchanged after surface modification (exp. P1a and P2a-c). The suspensions of cyclam-functionalized nanoparticles are remarkably stable without any detectable aggregation after more than one-year storage. On the other hand, the linkage of cyclam to negatively charged polymer nanoparticles results in a progressive aggregation (exp. P3a). Consequently, considering the colloidal stability of the cyclam-functionalized nanolatexes, it is highly preferable to perform post-functionalisation on nanoparticles resulting from DMPA-initiated polymerisations.
2.3 Metal-binding capacity and ligand accessibility
Owing to the well known high affinity of cyclam for cupric ions and according to our previous study on metal-complexing nanoparticles prepared by a one-step copolymerisation procedure (Fig. 1, pathway A), the metal binding ability of the particles is readily deduced from copper elemental analyses and spectrophotometric measurements [11].
In the presence of cupric ions, the cyclam-functionalized suspensions turn violet with a maximum absorption at 536 nm (ε = 134 l mol–1 cm–1): this absorption wavelength is similar to those observed with cyclam-functionalized nanoparticles obtained by the one-step copolymerisation of the polymerisable ligand 2 and is characteristic of monosubstituted cyclam-copper complexes [11]. Thus, the linkage of cyclam to the reactive nanoparticles involves only one nitrogen atom per cyclam.
Taking advantage of the transparency of the suspensions, the amount of copper–cyclam complexes is easily determined spectrophotometrically in good agreement with copper elemental analysis (exp. P2a, Table 2) [11]. Complexation experiments performed under stoichiometric conditions or in the presence of an excess of copper clearly illustrate the binding capacity and the ligand accessibility. Whatever the starting suspension, the complexation of the cyclam residues is quantitative (within the experimental limits, more than 95%). The copper contents in the resulting particles reach up to 0.28 mmol g–1 (Table 2).
Remarkably, the particles size remains unchanged after complexation. Furthermore, nanolatexes containing more than 0.2 mmol of cyclam-copper complex per gram of polymer are readily purified by dialysis without destabilization affording very stable transparent violet surfactant-free suspensions of 15–17 nm functionalized nanoparticles containing up to about 400 copper moieties per particles. Electrostatic stabilization arising from ionic repulsions between the positively charged Cu–cyclam functionalized nanoparticles accounts for the colloidal stability.
According to our previously published study [11], the ligand accessibility can be deduced from spectrophotometric titrations, which allow one to differentiate between two complexation processes: a rapid solution-like complexation which involves accessible cyclam residues located near the surface and a slower diffusion limited complexation process which involves cyclam residues entrapped within the core of the particles. Upon progressive addition of a dilute 0.01 M solution of copper nitrate, the suspensions of cyclam-functionalized particles instantaneously turn violet indicating that complexation readily takes place even at very low copper concentrations (Fig. 3 ). The nanoparticles act as sensors for cupric ions with a detection limit of about 5 × 10–4 mol l–1. The absorbance of the copper–cyclam complex linearly increases up to a maximum value that corresponds to the instantaneous complexation of all the accessible cyclam moieties in dilute medium. As illustrated in Fig. 3, in the case of 17-nm cross-linked particles (P2a), nanoparticles resulting from post-functionalisation exhibit a remarkable ligand accessibility: about 90–95% of the cyclam residues are involved in a rapid and quantitative solution-like complexation process. For all the suspensions prepared by post-functionalisation, complete cyclam complexation is achieved in the presence of stoichiometric amounts of copper at the equilibrium in dilute medium (overall Cu concentration 5 × 10–3 mol l–1). Titration experiments conducted with more concentrated solutions of copper nitrate (0.5 M) show that instantaneous complexation of all the cyclam residues readily occurs at the minute time scale in the presence of stoichiometric amounts of Cu and an overall copper concentration of about 5 × 10–2 mol l–1.
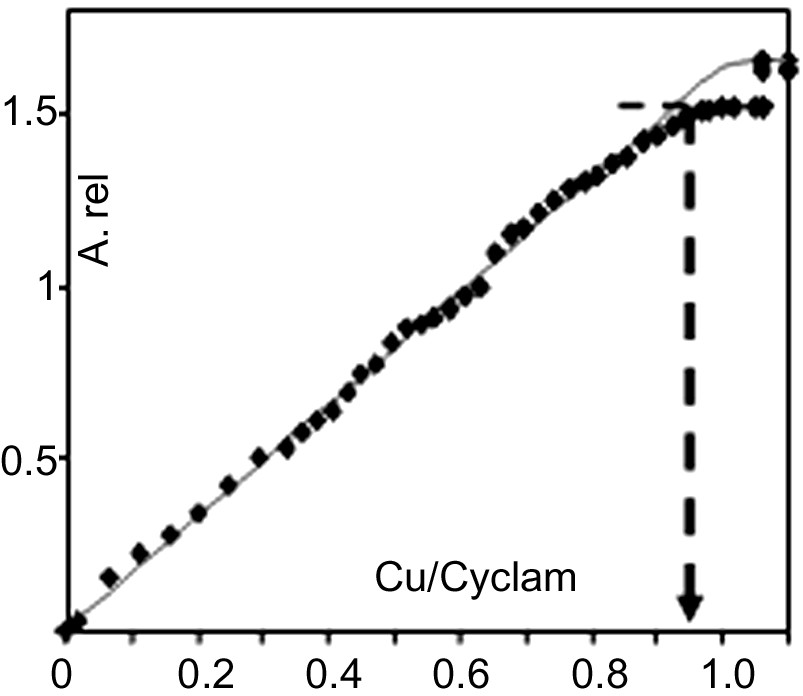
Spectrophotometric titration of Cu–cyclam complex upon progressive addition of dilute 0.01M Cu(NO3)2 to suspension P2a (nanoparticles average diameter: 17 nm, cyclam content 0.28 mmol g–1). Instantaneous relative absorbance (per gram of suspension) vs equiv. Cu added per cyclam. Filled squares: experimental; line: calculated (ε = 134 l mol–1 cm–1).
During the post-functionalisation step, the cyclam residues are preferentially grafted at the surface of the particles. This account quite well for the remarkable ligand accessibility of these cyclam post-functionalized nanoparticles that exhibit a real ‘solution-like’ behaviour comparable to classical soluble supramolecular structures [11,17,18]. As already mentioned above, for nanoparticles prepared by a one-step copolymerisation procedure (Fig. 1, pathway A), the cyclam residues are distributed between the core and the outer sphere of the particles. The ligand accessibility mainly depends on the size of the particles. For ultra-fine cross-linked particles in the 12–13-nm range, 75% of the whole cyclam residues are readily accessible for a rapid solution-like complexation process and almost complete complexation is reached at the equilibrium. For larger particles, in the 15–20-nm range, the surface-to-volume ratio decreases: only 40–50% of the cyclam residues exhibit a solution-like behaviour and the maximum complexation yield is about 85% [11].
Thus, the two-step synthetic procedure seems to be a useful alternative to prepare metal-complexing nanoparticles with high ligand accessibility and efficiency, which do not depend on the particle size. On the other hand, the cyclam content is limited by the post-functionalisation yield. Consequently, the copper binding capacity, 0.2–0.28 mmol g–1, is poor compared with nanoparticles resulting from a one-step copolymerisation procedure (0.6–0.65 mmol g–1) [11].
3 Conclusion
The results reported in this paper show that polymerisation of a reactive monomer in microemulsions followed by post-functionalisation allows the synthesis of ligand-functionalized nanoparticles in the 15–25-nm diameter range. The post-functionalisation of benzylhalide-functionalized nanolatexes permits the covalent binding of a selective azamacrocycle ligand like cyclam under very mild conditions. This procedure is hoped to be quite general and offers the opportunity to prepare nanoparticles functionalized with various selective ligands and adjustable selectivities thus allowing one to control concurrently the size and the selectivity. Emphasis must be put on the remarkable ligand accessibility and efficiency on nanoparticles resulting from surface post-grafting. Further investigations are currently being developed using other post-functionalisation conditions in order to increase the ligand load and the resulting binding capacity.
4 Experimental section
The microemulsions were prepared by adding dropwise the desired amount of mixture of monomers to a 15-wt% aqueous solution of DTAB. The solution was stirred until a clear transparent microemulsion was obtained. For dimethoxyphenyl acetophenone (DMPA) initiated polymerisation: DMPA (0.05 mol mol–1 of monomer) was introduced in the mixture of monomers before the preparation of the microemulsion. The freshly prepared microemulsion was transferred into a refrigerated flask and degassed with nitrogen for 20 min. The polymerisation was carried out under white light irradiation (2 × 100 W) with gentle stirring at room temperature under nitrogen overnight. For ammonium persulphate ((NH4)2S2O8)/tetramethyldiaminomethane initiating system: the microemulsion was transferred into a three-necked flask and degassed with nitrogen for 20 min. Ammonium persulphate (0.01 mol/mol of monomer) diluted in the minimum of water and tetramethyldiaminomethane (0.03 mol mol–1 of monomer) were successively added. The polymerisation was carried out at room temperature under nitrogen and with gentle stirring overnight.
The post-functionalisations were performed by adding the desired amount of cyclam directly into the crude suspension resulting from previous polymerisation. The mixture was stirred at room temperature for three days. The excess of cyclam was then removed by dialysis through a porous cellulose membrane (MWCO 3500) toward an aqueous solution of DTAB (15 wt%).
To separate and purify the resulting polymer, an excess of methanol was added to the suspension (2:1 v/v) and the precipitated polymer separated by centrifugation. The resulting white precipitate was dispersed in demineralised water (100 ml) and stirred overnight at 60 °C. This washing was made twice again and the polymer was then dried at 50 °C until its weight remained constant.
Spectrophotometric titrations were performed on a PerkinElmer spectrophotometer equipped with a reflection sphere by adding small aliquots (50–100 μl) of a 0.01 M aqueous solution of copper nitrate to the suspension (2 g) placed in a quartz cell. The absorbance at 536 nm was measured 3 min after each addition. The equilibrium binding capacity was obtained by adding an excess of copper nitrate to the suspension. After 24 h, the excess of cupric ions was removed eliminated by dialysis; the copper content was then deduced from the absorbance of the suspension at 536 nm and from the copper analysis of the polymer purified as described above.
Acknowledgements
We gratefully acknowledge Sylvain Desert (SCM, CEA Saclay, France) for QELS experiments and useful discussions.