1 Introduction
This account is intended to discuss the recent development [1–4] of a special class of organic ligands for colloidal nanocrystals, dendron and dendron-box ligands. Dendron and dendron-box ligands are evidently more complicated than the traditional single-chain organic ligands but simpler than the polymer based stabilizers. The instability of colloidal nanocrystals is a critically important and generally existing issue in the field of colloidal nanocrystals. We will discuss why dendron and dendron-boxes are rational solutions for the problem of instability. The performance of the resulting dendron-nanocrystals and box-nanocrystals will also be discussed. Discussion of the results will be limited to the surface modification of pre-formed nanocrystals with dendron and dendron-box ligands, although there is considerable ongoing effort on the synthesis with dendron ligands or dendrimers [5–9]. It is noticed that, up to the present, the size and size distribution of the nanocrystals directly synthesized using dendrons and dendrimers as the ligands [5–9] are significantly worse than those synthesized using traditional ligands.
The unique size-dependent properties of colloidal nanocrystals are of great interest for both fundamental studies and potential applications [10–12]. However, many current research activities are hindered by the unavailability of highly stable nanocrystals. Light-emitting diodes (LEDs) based on semiconductor nanocrystals have short lifetime, likely a result of the dissociation of the organic ligands from the nanocrystals due to the thermal effects of the devices in operation [13]. Detachment of the organic ligands from the nanocrystals exists as a major problem in using semiconductor nanocrystals for biological labeling [1,2]. The enhancement effect of magnetic nanocrystals for magnetic resonance imaging [14,15] is still in its infancy because of the instability of the ligands on the surface of the nanocrystals. Thus, it is important to find a general method to solve the critical instability problem of colloidal nanocrystals. This research group initiated a systematic effort in this direction, and the results obtained so far will be summarized in this account.
The studies of photochemical instability of thiol-coated nanocrystals are introduced in Section 2. Guided by the mechanism of photochemical instability, the work on using dendron ligand to stabilize nanocrystals is described in Section 3. In Section 4, further work to form a dendron-box around each nanocrystal is described. The superior chemical, photochemical, and thermal stability of the box-nanocrystals will also be discussed. A small section (Section 5) briefly discusses potential applications of box-nanocrystals.
2 Photochemical instability of thiol-coated nanocrystals
Colloidal nanocrystals are metastable species compared to the corresponding bulk crystals. Typically, these metastable species need to be kinetically stabilized by a monolayer of organic ligands (or called stabilizers, capping groups, surfactants, etc.). Thiol ligands are widely used for semiconductor and noble metal nanocrystals because of the relatively strong bonding between thiols and the surface heavy metals of the nanocrystals. Unfortunately, even for these strongly bonded nanocrystal–ligand complexes, the instability has still been observed as a problem. Systematic studies were conducted by our group to reveal the mechanisms [1,16]. There are some significant similarities between the semiconductor nanocrystals and noble metal nanocrystals, and only the results for semiconductor systems will be discussed below [1].
Hydrophilic thiols with different structures were chosen to modify the nanocrystals following a known procedure. The complete displacement of original ligands and the removal of free ligands from solution were monitored by nuclear magnetic resonance (NMR) spectroscopy. The NMR distinction of free ligands and the corresponding bonded ligands is based on the fact that the peaks of the bonded ligands are significantly broader than those of the free ligands due to the close packing nature of the surface ligands on nanocrystals. The photooxidation of the CdSe nanocrystal core was monitored by UV–Vis spectroscopy. Due to quantum confinement, the absorption spectrum of the semiconductor nanocrystals with a given composition shifts to blue as the size of the nanocrystals decreases. This provides a convenient and sensitive probe for any etching process caused by photooxidation or other means. The experimental results of the studies are briefly summarized as follows.
Experimental results revealed that the main instability process for thiol-coated CdSe nanocrystals is photooxidation, which occurs in three distinguishable stages. The initiation stage, also the most critical stage, is the photocatalytic oxidation of thiol ligands. The structure of the ligands was found to play an important role in this stage. The longer and more flexible the ligands were, the slower the photocatalytic process. The product of the photocatalytic oxidation of the thiol ligands was identified as disulfides, which detached from the surface of the nanocrystals upon formation. If free ligands were available, they would bind to the surface of the partially uncoated nanocrystals generated by the photocatalytic process. If no free thiol ligands were in the solution, the nanocrystals were found to precipitate from the solution if the disulfides were soluble in the solution. Insoluble disulfides tended to form a micelle-like structure around the semiconductor nanocrystals, which temporarily stabilize the nanocrystals. After all thiols were converted to insoluble disulfides, the photocatalytic process would stop and the photooxidation of the semiconductor nanocrystals themselves started. When the size of the nanocrystals shrunk to a certain value, the micelle-like structure would crash and precipitation of nanocrystals was observed. Fig. 1 illustrates the mechanisms discussed above.
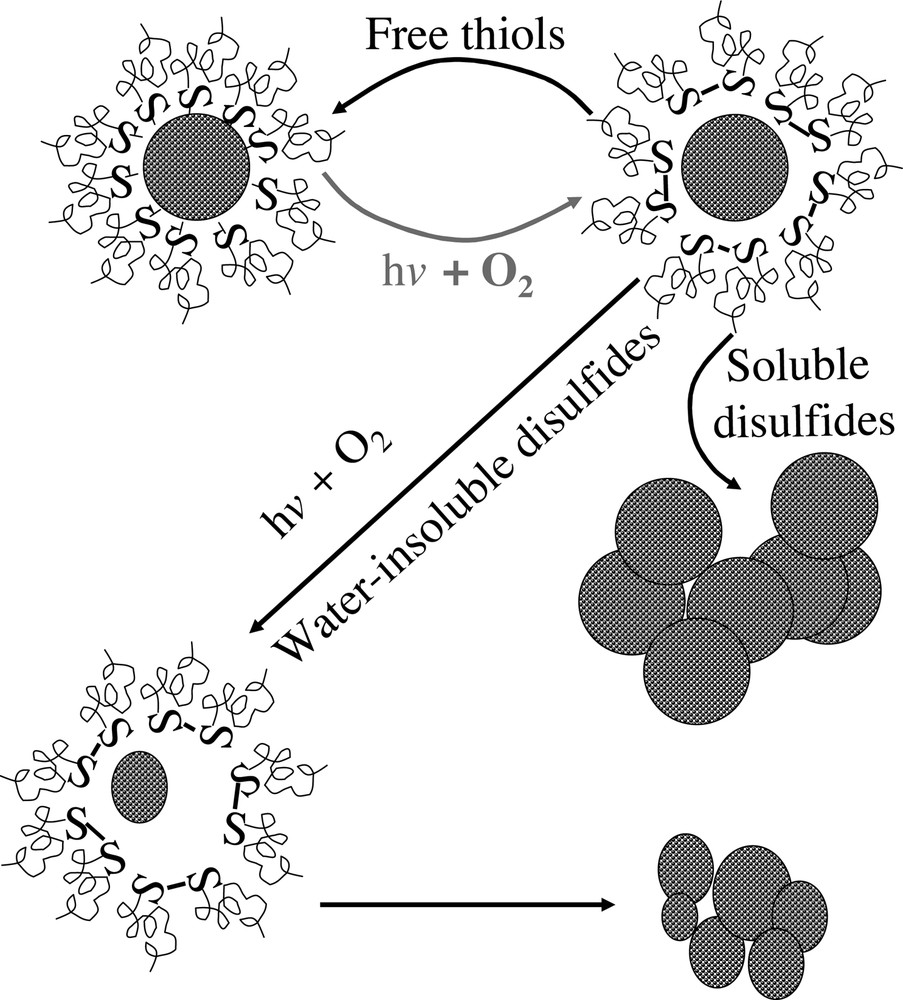
Mechanisms of photooxidation of thiol-coated semiconductor nanocrystal–ligands complexes. The initial – also the most critical – step is illustrated in light gray.
The experimental results further demonstrate that the initial step of the photooxidation process of thiol-coated semiconductor and noble metal nanocrystals (shown in red in Fig. 1) is the key step to determine the lifetime of the colloidal suspension. Because photoradiation and an oxygen environment cannot be avoided in many cases, it is essential to develop new types of ligands that can form a monolayer of organic coating on the surface of each nanocrystal to slow down the diffusion of oxygen molecules.
3 Dendron-nanocrystals
The diffusion of activated oxygen species and other small molecules through the ligand monolayer around each nanocrystal can vary substantially by changing the structure of the ligands. Evidently, a thick ligand shell dramatically improves the stability of the nanocrystals by slowing down the diffusion of the small molecules. However, in many cases, the physical size of the ligand monolayer needs to be as thin as possible. For instance, the physical penetration of nanocrystals in biological systems will dramatically be affected by the size of the nanocrystal–ligand complex. In another example, the charge transport between nanocrystals in optoelectronic devices was found to be strongly dependent on the thickness of the ligand monolayer. All these considerations motivated us to consider the possibility of building up an ultra-thin but extremely dense ligand monolayer on the surface of each nanocrystal.
Organic dendron molecules are those hype and regularly branched molecules, which are considered to be proper substitute ligands for the conventional single-chain ligands for three reasons. First, steric crowd characteristics of organic dendrons may provide a closely packed but thin ligand layer. Importantly, the natural cone shape of a dendron is ideal to fill the spherical ligand shell layer of nanocrystals (Fig. 2 ). Second, the inter- and intra-molecular chain tangling of the dendrons with relatively flexible branches may further slow the diffusion of small molecules or ions from the bulk solution into the interface between a nanocrystal and its ligands. Third, the multiple peripheral groups of dendrons could be further close-linked to put the nanocrystals into a sealed shell, or serve as the function group to conjugate the nanocrystals with other molecules.
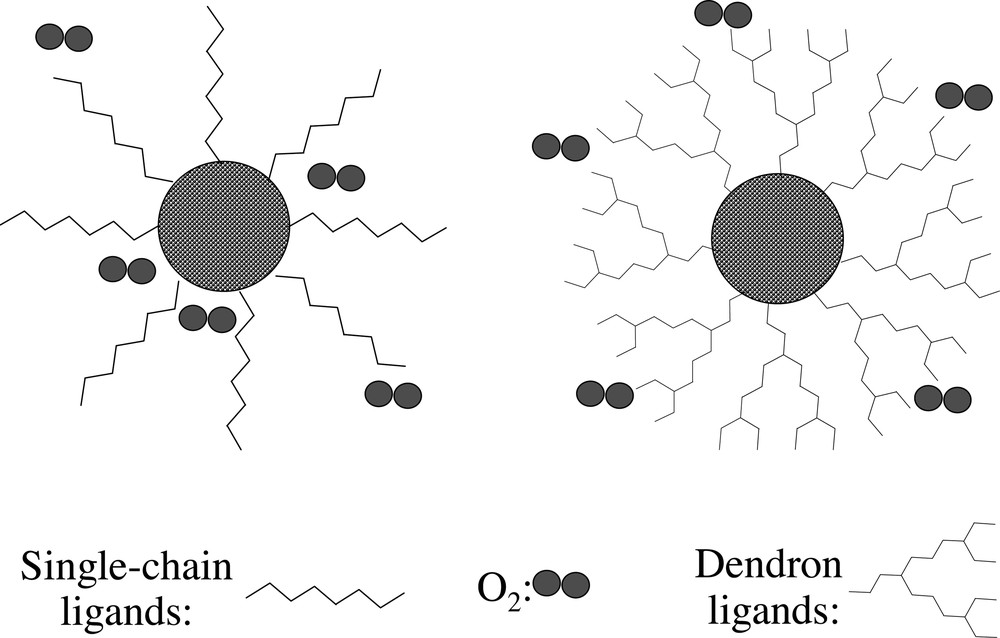
Schematic demonstration of the comparison of nanocrystals coated with different types of organic ligands.
The focal point of the dendron molecules was chosen as the anchoring point to the surface of the nanocrystals in our design, and the periphery should be flexible for maximum interactions between the branched chains [2]. As expected, the dendron-nanocrystals are significantly more stable than the nanocrystals coated by traditional single-chain ligands with similar molecular mass. In addition, the photochemical stability of the dendron-nanocrystals was found to strongly depend on the number of generations of the dendron ligands (Fig. 3 ). As illustrated in Fig. 3, generation 3 (G3) dendron-nanocrystals did not show any significant optical change for about 40 hours under photooxidation conditions. Without UV radiation, the aqueous solutions of G2 and G3 dendron-nanocrystals after removing excess ligands can be stored under ambient conditions for several months without any precipitation.
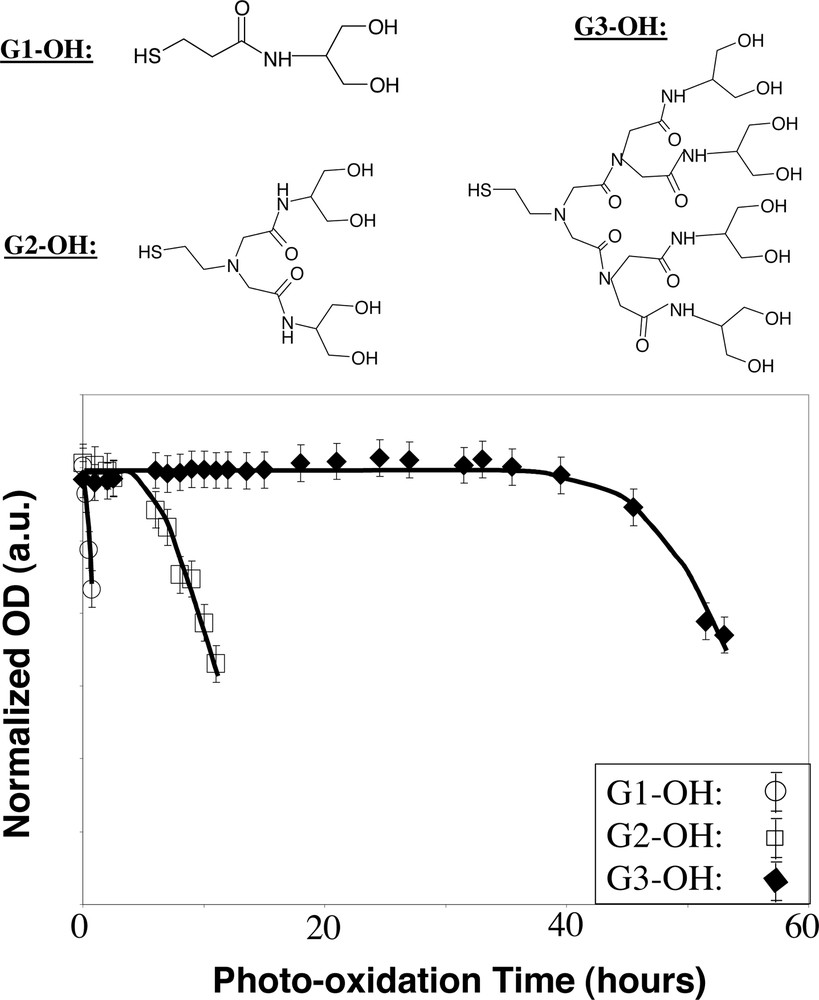
Photochemical stability of dendron-nanocrystals under UV radiation in air. The structures of the dendron ligands are illustrated on the top panel.
The thermal and chemical stability of the dendron-coated nanocrystals are also significantly better than the nanocrystals coated with traditional single-chain ligands. The improved overall stability of the dendron-nanocrystals make it possible to withstand the coupling reactions needed for the chemical and biochemical functionalization of nanocrystal–ligands complexes. For example, the amide formation on the outer surface of the dendron-nanocrystals through either ester–amine coupling (Fig. 4 ) or the traditional EDC coupling worked well.
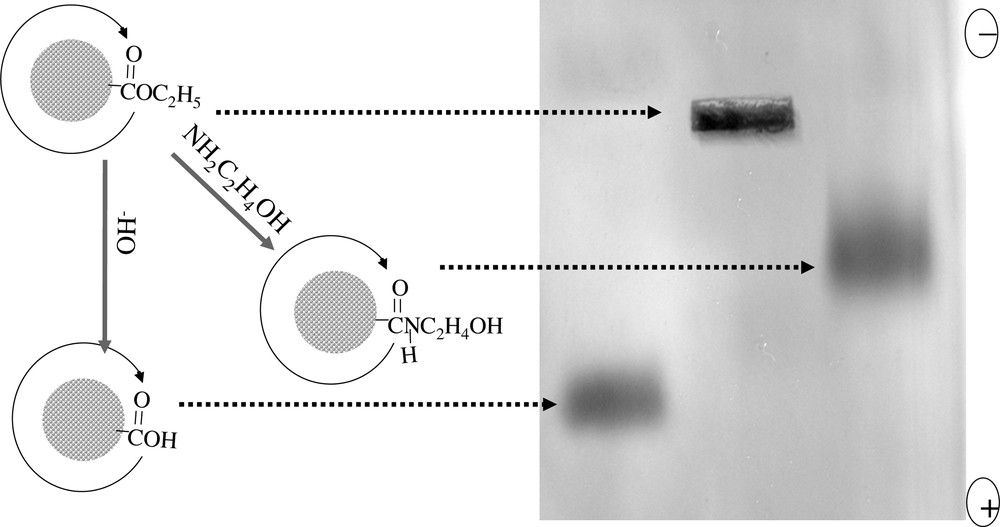
Gel-electrophoresis of CdSe dendron-nanocrystals. Middle: nanocrystals coated by ester-terminated dendron ligands (insoluble in water and not moved); left: after the hydrolysis of the ester groups; right: after the conversion of the esters to amide.
Unlike the semiconductor nanocrystals coated by regular single-chain ligands, CdSe dendron-nanocrystals can run through a chromatography column as a tight band. The popular separation technique in biological sciences, electrophoresis, also worked for CdSe dendron-nanocrystals. As shown in Fig. 4, CdSe dendron-nanocrystals with different functional groups on their outer surface show significantly different mobility on a gel plate. Similar results have been observed for noble metal dendron-nanocrystals [2].
The dendron-nanocrystals had the same solubility as the dendron ligands. The G3-OH dendron nanocrystals were soluble in polar solvents, such as water and DMSO. The absorption spectra of the nanocrystals did not change upon ligand exchange. For noble metal dendron-nanocrystals, a similar improvement of their photochemical and thermal stability was also observed. The photoluminescence (PL) of the CdSe core nanocrystals is completely quenched, as previously observed for nanocrystals coated with thiol ligands. However, the band-edge PL of CdSe/CdS core/shell dendron-nanocrystals partially remained, about 20% of the original value.
4 Box-nanocrystals
The substantial improvement of the photochemical and thermal stability of the dendron-nanocrystals (Fig. 3) is very encouraging. In order to further slow down the diffusion of small molecules through the ligand monolayer, we explored the global cross-linking of all dendron ligands to form a dendron-box around each nanocrystal (box-nanocrystal). We hypothesized that the targeted cross-linking will make the mean free length of diffusion extremely small. As a result, the penetration of oxygen molecules through the ligand monolayer may become practically impossible.
The structure of dendron ligands is ideal for the desired global cross-linking because each dendron ligand possesses multiple terminal groups. For instance, a G3 dendron can easily be designed to have eight functional groups on the outer surface. However, a global cross-linking only requires two to three bonding sites per dendron to its neighbors in the ligand monolayer. A flexible interior and periphery of the dendron ligands may provide a great deal of inter-ligand chain tangling. As a result, inter-ligand cross-linking, instead of intra-ligand cross-linking, may readily occur in the ligand monolayer of each particle. The enhanced stability of the dendron-nanocrystals discussed in the above section will warrant that the desired cross-linking reactions will not destroy the nanocrystal–ligand complexes.
Two different strategies have been developed by our group for the formation of box-nanocrystals (Fig. 5 ). The top scheme [3] in Fig. 5 is based on ring-closing-metathesis (RCM) reactions, and the dendron ligands were chosen to be terminated with carbon–carbon double bonds. The resulting box-nanocrystals were soluble in typical organic solvents, such as benzene, toluene and chloroform. The second strategy, dendrimer-bridging, [4] was designed to directly yield water-soluble box-nanocrystals. The amine groups on the outer surface of the box-nanocrystals were found to be chemically and biochemically accessible (see details below).
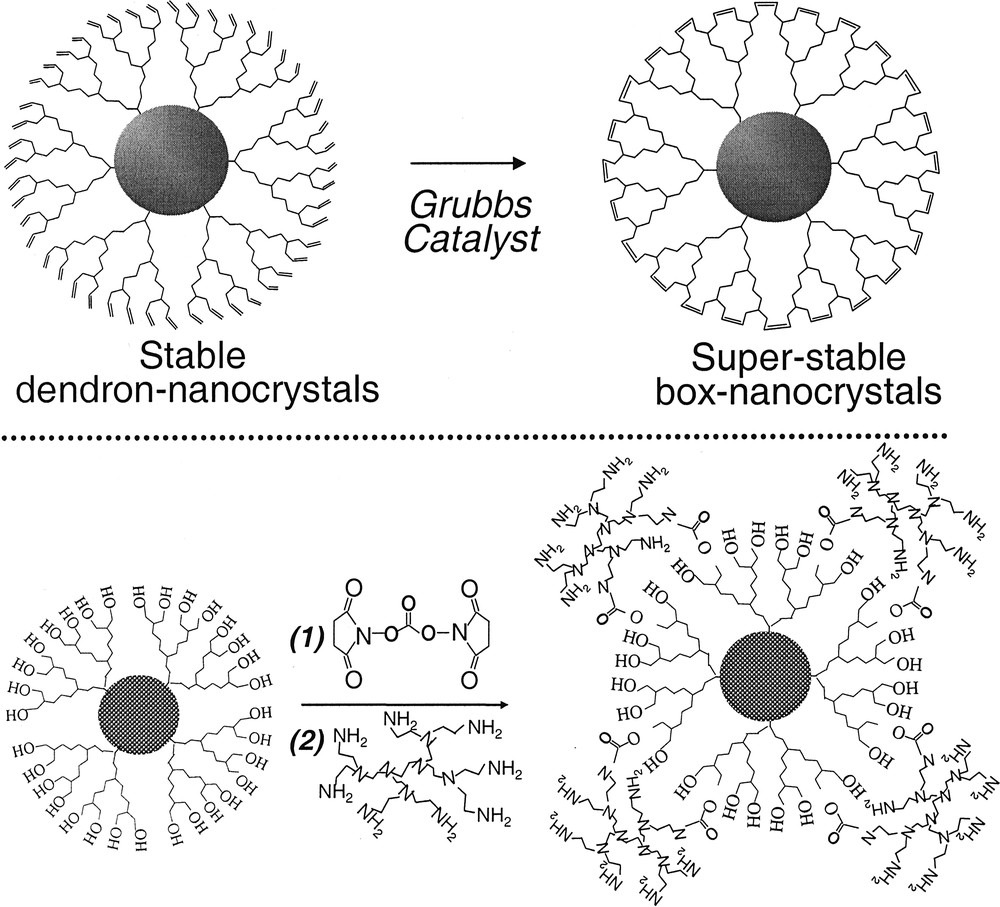
Schematic demonstration of two strategies for the formation of box-nanocrystals. Top: RCM route. Bottom: dendrimer-bridging route.
The formation of the box-nanocrystals through both approaches has been well documented. [3] Briefly, transmission electron microscope (TEM) results revealed that the cross-linking was successfully limited to an intra-particle manner. NMR and IR spectra revealed that cross-linking reactions went very efficiently, and the global cross-linking required for the formation of dendron-boxes was demonstrated by both NMR and mass spectroscopy. The cross-linking process did not affect either PL or absorption properties of the semiconductor dendron-nanocrystals.
However, the solubility of the nanocrystals after the cross-linking varied from that of the original dendron-nanocrystals [3]. The original dendron-nanocrystals terminated by carbon–carbon double bonds were soluble in typical non-polar organic solvents, but the corresponding box-nanocrystals were only soluble in aromatic solvents or polar organic solvents, such as benzene, DMSO, and DMF. For the dendrimer-bridging route, the resulting box-nanocrystals became insoluble in basic aqueous solution although the –OH terminated dendron-nanocrystals were extremely soluble under the same conditions. These changes are considered to be consistent with the variation of the surface functional groups on the outer surface of the nanocrystal–ligand complexes.
The stability of the box-nanocrystals against harsh chemical, thermal and photochemical treatments was proven to be superior in comparison to that of the corresponding dendron-nanocrystals [3,4]. Fig. 6 illustrates the superior photochemical stability of CdSe/CdS core/shell box-nanocrystals synthesized by the RCM route (top panel) and the excellent stability against acid etching of the CdSe/CdS core/shell box-nanocrystals obtained via the dendrimer-bridging route (bottom panel). For comparison, the results of the corresponding dendron-nanocrystals are also shown in Fig. 6. In the bottom panel, the stability results for the locally cross-linked dendron-nanocrystals formed by the use of either a diamine or a trisamine linker are also illustrated. The chemical stability against strong oxidants, such as H2O2, and the thermal stability against high-temperature (100 °C) sintering of the semiconductor box-nanocrystals were also found to be superior in comparison to the corresponding dendron-nanocrystals [3,4].
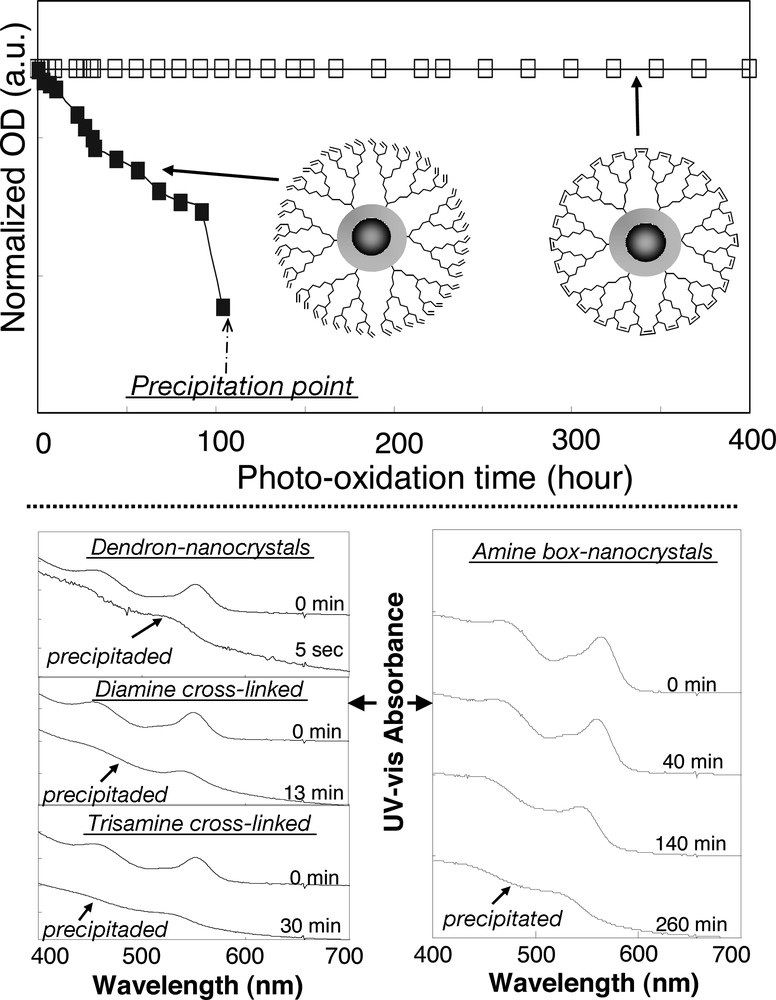
Top: photochemical stability of the CdSe/CdS core/shell box-nanocrystals formed by the RCM route. Bottom: chemical stability of CdSe/CdS core/shell box-nanocrystals formed through the dendrimer-bridging route in HCl solutions (pH = 1).
The superior stability of the box-nanocrystals in comparison to the corresponding original dendron-nanocrystals and the locally cross-linked dendron-nanocrystals (see Fig. 6 as examples) verifies the original hypothesis mentioned in the first paragraph of this section, that the mean free length of diffusion of small molecules, including activated oxygen species, hydrogen ions, hydrogen peroxide, etc, has been significantly suppressed by the global cross-linking of all dendron ligands on the surface of each nanocrystal.
5 Applications
Dendron-nanocrystals and box-nanocrystals, especially the latter, are of great interest for several ongoing efforts, such as biomedical labeling, LEDs, and lasers, as well as some potential new directions. In this section, we discuss two examples, one for an ongoing application and one for a potential direction.
The superior stability of the box-nanocrystals makes it possible to readily couple chemical and biochemical functions onto the outer surface of the box-nanocrystals. These functionalized box-nanocrystals are ideal species for quantitative and reproducible studies using colloidal nanocrystals. For example, biotin molecules were successfully coupled onto the surface of the box-nanocrystals made through the dendrimer-bridging route. With these biotinated box-nanocrystals, quantitative and reproducible detection of a trace amount of avidin was demonstrated (Fig. 7 , top). The control experiments shown in Fig. 7 (top) indicate that no nonspecific bonding existed and the biotinated box-nanocrystals were stable in the desired bio-environment [4].
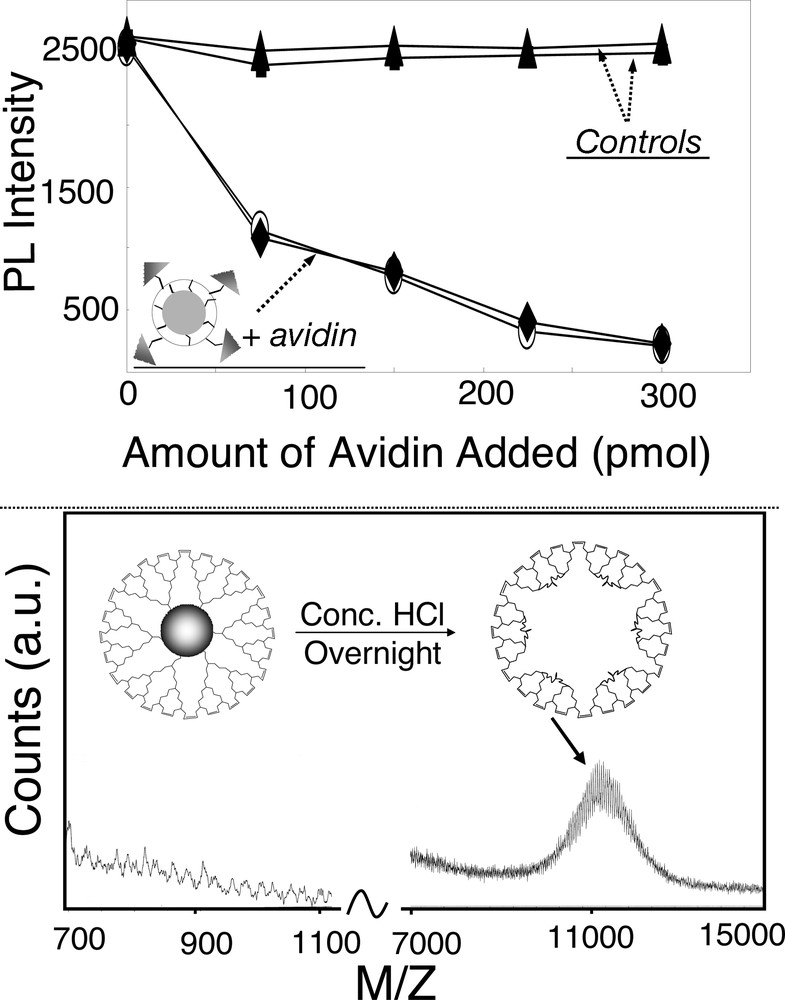
Top: quantitative and reproducible detection of avidin by biotinated box-nanocrystals. Two control experiments: (1) biotinated box-nanocrystals in plain PBS buffer and (2) original box-nanocrystals plus avidin in PBS buffer. Bottom: formation and detection of empty dendron-boxes.
The second example takes advantage of the monodisperse and nanometer-sized nature of the core semiconductor nanocrystals [3]. It is an active field of study to synthesize mesoporous cavities in solution for drug delivery, catalysis, etc. We demonstrated that the dendron-box retained its integrity after the inorganic nanocrystal core was removed by etching the box-nanocrystals in concentrated HCl solution overnight (Fig. 7, bottom). Mass spectroscopy data revealed that the size distribution of the resulting mesoporous dendron-boxes was within 10%, similar to that of the original nanocrystals. These empty boxes are not only nearly monodisperse, but also have a very thin wall. The potential applications of such cavities are in active consideration.
6 Conclusion and perspective
The impressive stability of the dendron-nanocrystals and box-nanocrystals is encouraging. Our preliminary results indicate that this is a versatile solution to a common issue for colloidal nanocrystals of nearly all compositions – instability during the chemical and biochemical manipulation and device operation. The generality of this solution is due to the general underlying principle, minimizing the mean free length of diffusion of small molecules in the ligand monolayer around each nanocrystal.
As discussed above, this general solution was originated from a systematic and quantitative study of the photochemical instability of thiol-coated semiconductor and metal nanocrystals [1,16]. Because fundamental knowledge of surface and ligand chemistry of colloidal nanocrystals is still scarce, more fundamental research is needed and such efforts will be continuously proven to be rewarding.
The structures of the interior of the dendrons employed so far have been mostly based on availability. It would be ideal that one could use dendron ligands that are specifically designed for surface modification of colloidal nanocrystals. It is anticipated that the interior structure of the dendrons may vary from application to application. For instance, biocompatibility will not be an issue for optoelectronic devices.
Acknowledgements
Financial support from the NSF is highly appreciated.