1 Introduction
AlPO4-n are microporous crystalline aluminophosphates with open-framework structures similar to zeolites [1]. They are built up from strictly alternating corner-sharing aluminum and phosphorus tetrahedra. Their discovery was followed by the silicoaluminophosphates [2,3], titanium aluminophosphates [4], metal aluminophosphates [5–7] and metal silicoaluminophosphates [8], designated SAPO-n, TAPO-n, MeAPO-n and MeAPSO-n, respectively. These compounds are still a matter of interest because of their properties as catalysts and molecular sieves. Many of them possess original framework topologies that do not exist in the family of microporous silicates or aluminosilicates. On the other hand, some AlPOs, SAPOs or MeAPOs are structurally related to zeolites. For example AlPO4-17 [9], AlPO4-20 [10], AlPO4-34 [11] and SAPO-37 [3] are known to possess structures with the erionite (ERI), sodalite (SOD), chabazite (CHA) and faujasite (FAU) framework topology, respectively. The crystal system can be the same for the zeolite and the aluminophosphate analog but space groups generally differ. Indeed, the space group symmetry for disordered Si and Al in zeolites has to be reduced to account for the strict ordering of P and Al in the aluminophosphate. Moreover, extraframework atoms like O or F can be present in as-made compounds, thus decreasing the symmetry of the framework. As an example, the triclinic form of AlPO4-34 (CHA framework topology) can only be obtained in the presence of fluorine. In the as-made compound, two fluorine atoms bridge two Al atoms of a four-ring, connecting two D6R’s of the chabazite-like topology [12]. This leads to a distortion of the trigonal symmetry of chabazite, with space group P. Upon calcination, fluorine bridges are removed and the symmetry of the calcined material becomes rhombohedral, but the space group is R, which is still lower than the space group R of the zeolite.
Extraframework oxygen atoms, generally in the form of OH– groups, can also bridge aluminum atoms, leading to five-coordinate aluminum species. Such oxygen bridges have been found in several compounds, including AlPO4-17 [9] and AlPO4-18 [13]. In the particular case of AlPO4-17, oxygen species bridge aluminum atoms located in opposite six-rings, at the top and at the bottom of each cancrinite cage. These extraframework sites are partly occupied, all Al atoms in the six-rings being considered as forming, on average, one third of the time (or space) a tetrahedron and two thirds of the time (or space) a trigonal bipyramid. Each cancrinite cage thus contains two extraframework OH– groups, balancing the charge of the protonated template. In the case of a material prepared with piperidine, the unit cell formula is Al18P18O72·4(C5NH12+·OH–). Because of the disorder of extraframework hydroxyl species, no evidence was found for symmetry lower than hexagonal and the unit cell was described in space group P63/m (P63/mmc for the corresponding zeolite) [9].
AlPO4-17 is generally synthesized under hydrothermal conditions in the presence of small cyclic amines like piperidine [9], quinuclidine [7,14] or cyclohexylamine [12,15]. However, Gao et al. [16] could also prepare AlPO4-17 from a non-aqueous medium using methylamine as template. Crystals are in the form of elongated hexagonal prisms, revealing the symmetry of the unit cell. Though their position has not been precisely determined, templating molecules are located in the large ellipsoidal cages of the structure. The distance between two organic molecules (piperidinium ions) in the prism corresponds approximately to half of the c-axis value, that is ≈ 7 Å [9]. Theoretically, it would then be possible to replace two piperidinium ions by a single molecule, for example a doubly protonated diamine, with a N–N distance close to 7 Å. Patents effectively report the synthesis of SAPO-17 materials using hexamethonium ions [2], but the structure of the corresponding solids has never been studied.
The present work reports on the synthesis, structure determination and characterization of AlPO-ERI, isostructural to AlPO4-17, and obtained in the presence of N,N,N′,N′-tetramethyl-1,6-hexanediamine (TMHDA). Moreover, the structure of the calcined rehydrated compound is also reported.
2 Experimental section
2.1 Synthesis
AlPO-ERI was synthesized under hydrothermal conditions in the presence of TMHDA as template. Aluminum isopropoxide Al(OiPr)3 (98+%, Aldrich) and phosphoric acid (85 wt.% in water, Prolabo) were used as aluminum and phosphorus sources, respectively. Typically, 14.7 g of aluminum isopropoxide were dispersed in 30 ml of water and the suspension was stirred for 2 h. Then 4.92 ml of H3PO4 were added dropwise and the obtained gel was stirred for an additional hour. Finally, 7.75 ml of diamine were slowly added and stirring was maintained for an additional 2 h. The resulting gel, with the composition Al2O3–P2O5–TMHDA–46 H2O was transferred into a Teflon-lined stainless steel autoclave and heated at 200 °C for 60 h. The solid, recovered by filtration, appeared in the form of elongated hexagonal prisms. It was washed with distilled water and air-dried at room temperature. Part of the crystals was calcined in air at 500 °C for 24 h. After cooling down the temperature, the calcined AlPO-ERI was rehydrated under ambient atmosphere for 15 days.
2.2 X-ray data collection and structure determination
The crystals from the as made material are invariably twinned as manifested when viewed under the polarizing microscope. Therefore an attempt was made to cut a single crystal from such twins.
From both samples (as made and calcined/rehydrated) a single crystal data set was collected at room temperature with a Picker 4-circle diffractometer with Cu Kα radiation. The structure was solved using SHELX-86 [17] and refined with SHELX-93 [18]. Further details of the data collection and the crystallographic data are given in Table 1. The crystal from the as made material still showed some twinning and it was necessary to apply a twin refinement with restrained anisotropic displacement parameters. In addition, restraints on bond distances and bond angles had to be applied to the template molecule in the as made material. Selected interatomic distances and bond angles are reported in Table 2.
Crystal data and structure refinement for AlPO-ERI
Compound name | AlPO-ERI as made | AlPO-ERI, rehydrated |
Empirical formula | |C10 H26 N2, 2 OH| [Al9P9O36] | |17 H2O| [Al9P9O36] |
Formula weight | 1305.89 | 1403.82 |
Space group | P2(1) (No. 4) | P2(1)/n (No. 14) |
Z | 2 | 4 |
a (Å) | 13.163(14) | 13.283(17) |
b (Å) | 14.793(15) | 14.910(14) |
c (Å) | 13.215(14) | 22.76(3) |
β (°) | 119.74(8) | 90.19(10) |
λ (Å) | 1.54178 | 1.54178 |
Crystal size (mm) | 0.1 × 0.1 × 0.1 | 0.1 × 0.1 × 0.1 |
θ range (°) | 3.85–50.00 | 3.54–49.97 |
Index ranges | –13 ≤ h ≤ 11, 0 ≤ k ≤ 14, 0 ≤ l ≤ 13 | –13 ≤ h ≤ 13, –14 ≤ k ≤ 0, –22 ≤ l ≤ 0 |
Number of reflections (> 2 σ(I)) | 1687 | 2981 |
Number of reflections | 2403 | 4616 |
Number of structural parameters | 624 | 668 |
Number of restraints | 509 | 18 |
R(F2) for all/> 2 σ(I)) reflections | 0.0845/0.0628 | 0.1268/0.0767 |
Rw(F2) for all//> 2 σ(I)) reflections | 0.1915/0.1627 | 0.2245/0.1986 |
Refinement method | Full-matrix LS on F2 | Full-matrix LS on F2 |
Interatomic distances (Å) and bond angles (°) of as made AlPO-ERI
P(1)–O(1) | 1.50(2) |
P(1)–O(3) | 1.51(2) |
P(1)–O(2) | 1.513(12) |
P(1)–O(4) | 1.524(13) |
P(2)–O(5) | 1.501(12) |
P(2)–O(8) | 1.507(13) |
P(2)–O(7) | 1.51(2) |
P(2)–O(6) | 1.53(2) |
P(3)–O(11) | 1.43(2) |
P(3)–O(12) | 1.504(11) |
P(3)–O(10) | 1.549(14) |
P(3)–O(9) | 1.553(17) |
P(4)–O(16) | 1.43(2) |
P(4)–O(15) | 1.469(16) |
P(4)–O(13) | 1.52(2) |
P(4)–O(14) | 1.568(19) |
P(5)–O(20) | 1.41(3) |
P(5)–O(17) | 1.484(18) |
P(5)–O(19) | 1.49(2) |
P(5)–O(18) | 1.58(2) |
P(6)–O(22) | 1.47(2) |
P(6)–O(21) | 1.48(2) |
P(6)–O(23) | 1.50(2) |
P(6)–O(24) | 1.531(17) |
P(7)–O(25) | 1.452(19) |
P(7)–O(26) | 1.52(2) |
P(7)–O(28) | 1.54(2) |
P(7)–O(27) | 1.57(2) |
P(8)–O(29) | 1.44(2) |
P(8)–O(32) | 1.53(3) |
P(8)–O(30) | 1.54(2) |
P(8)–O(31) | 1.56(3) |
P(9)–O(35) | 1.47(2) |
P(9)–O(34) | 1.51(2) |
P(9)–O(33) | 1.54(2) |
P(9)–O(36) | 1.57(2) |
Al(1)–O(31) | 1.67(3) |
Al(1)–O(5) | 1.715(12) |
Al(1)–O(21) | 1.77(2) |
Al(1)–O(2) | 1.774(13) |
Al(2)–O(14) | 1.67(2) |
Al(2)–O(12) | 1.708(11) |
Al(2)–O(8) | 1.732(14) |
Al(2)–O(25) | 1.76(2) |
Al(3)–O(4) | 1.687(14) |
Al(3)–O(10) | 1.708(13) |
Al(3)–O(18) | 1.72(2) |
Al(3)–O(34) | 1.73(2) |
Al(4)–O(3) | 1.71(2) |
Al(4)–O(36) | 1.73(2) |
Al(4)–O(24) | 1.74(2) |
Al(4)–O(16) | 1.76(2) |
Al(4)–O(2H) | 2.24(6) |
Al(5)–O(17) | 1.714(19) |
Al(5)–O(32) | 1.72(3) |
Al(5)–O(13) | 1.76(3) |
Al(5)–O(7) | 1.77(2) |
Al(5)–O(3H) | 2.13(5) |
Al(6)–O(22) | 1.81(3) |
Al(6)–O(19) | 1.82(2) |
Al(6)–O(11) | 1.84(2) |
Al(6)–O(28) | 1.86(2) |
Al(6)–O(1H) | 1.96(3) |
Al(7)–O(27) | 1.68(2) |
Al(7)–O(29) | 1.73(2) |
Al(7)–O(1) | 1.76(2) |
Al(7)–O(20) | 1.94(3) |
Al(7)–O(2H) | 2.00(5) |
Al(8)–O(30) | 1.72(3) |
Al(8)–O(35) | 1.77(2) |
Al(8)–O(9) | 1.777(16) |
Al(8)–O(15) | 1.895(16) |
Al(8)–O(1H) | 1.91(2) |
Al(9)–O(33) | 1.70(2) |
Al(9)–O(6) | 1.72(2) |
Al(9)–O(26) | 1.74(3) |
Al(9)–O(23) | 1.81(2) |
Al(9)–O(3H) | 2.22(5) |
P(1)–O(1)–Al(7) | 139.3(13) |
P(1)–O(2)–Al(1) | 142.9(10) |
P(1)–O(3)–Al(4) | 141.0(14) |
P(1)–O(4)–Al(3) | 170.3(10) |
P(2)–O(5)–Al(1) | 170.3(11) |
P(2)–O(6)–Al(9) | 138.7(15) |
P(2)–O(7)–Al(5) | 137.7(15) |
P(2)–O(8)–Al(2) | 148.0(8) |
P(3)–O(9)–Al(8) | 131.2(10) |
P(3)–O(10)–Al(3) | 146.8(9) |
P(3)–O(11)–Al(6) | 133.5(13) |
P(3)–O(12)–Al(2) | 171.9(11) |
P(4)–O(13)–Al(5) | 143.0(13) |
P(4)–O(14)–Al(2) | 152.0(14) |
P(4)–O(15)–Al(8) | 140.1(10) |
P(4)–O(16)–Al(4) | 157.6(14) |
P(5)–O(17)–Al(5) | 146.1(15) |
P(5)–O(18)–Al(3) | 146.0(18) |
P(5)–O(19)–Al(6) | 141.8(13) |
P(5)–O(20)–Al(7) | 134.4(19) |
P(6)–O(21)–Al(1) | 147.5(12) |
P(6)–O(22)–Al(6) | 143.4(14) |
P(6)–O(23)–Al(9) | 144.2(16) |
P(6)–O(24)–Al(4) | 138.1(15) |
P(7)–O(25)–Al(2) | 150.6(14) |
P(7)–O(26)–Al(9) | 147.9(14) |
P(7)–O(27)–Al(7) | 148.2(13) |
P(7)–O(28)–Al(6) | 140.3(12) |
P(8)–O(29)–Al(7) | 151(2) |
P(8)–O(30)–Al(8) | 151.0(14) |
P(8)–O(31)–Al(1) | 147.1(17) |
P(8)–O(32)–Al(5) | 141(2) |
P(9)–O(33)–Al(9) | 141.9(14) |
P(9)–O(34)–Al(3) | 152.1(17) |
P(9)–O(35)–Al(8) | 148.9(14) |
P(9)–O(36)–Al(4) | 135.7(15) |
Al(8)–O(1H)–Al(6) | 142.5(6) |
Al(7)–O(2H)–Al(4) | 143.9(15) |
Al(5)–O(3H)–Al(9) | 134.8(13) |
2.3 Characterization
Thermal analysis data were recorded on a Setaram TGDT A92 apparatus. Samples were heated in air from 25 to 750 °C at a heating rate of 5 °C/min.
SEM pictures were obtained on a Hitachi S800 microscope.
Solid-state magic angle spinning (MAS) NMR spectra were acquired on a Bruker DSX 400 spectrometer operating at 104.2 and 161.2 MHz for 27Al and 31P nuclei, respectively. Samples were spun at 11 kHz at the magic angle in a double-bearing 4 mm probe head. The pulse lengths were 2 μs (π/4) and 0.7 μs (π/6), and the recycle delays were 30 and 1 s for 31P and 27Al nuclei, respectively. 31P and 27Al chemical shifts were referenced to H3PO4 (85 wt.%) and Al(H2O)63+, respectively.
Two dimensional-five quanta (2D-5Q) 27Al MAS NMR spectra were collected at 14 kHz using the two-pulse sequence originally reported by Medek et al. [19] and further modified by Fernandez and Amoureux [20] for the study of S = 5/2 nuclei. The five quanta coherence was excited with a 2 μs pulse, corresponding to a radio frequency of 175 kHz. Three hundred twenty scans were accumulated for each free induction decay with a recycle delay of 500 ms. One hundred twenty-eight time domain real data points were acquired in the indirect dimension, and pure phase spectra were obtained using the TPPI scheme [21,22]. A shearing transformation was applied to the spectra in order to obtain isotropic projections parallel to the f1 direction.
27Al–31P CP/MAS experiments were carried out using a three-channel 1H-X-Y probe head and a conventional spin lock sequence with a spin-locking field of 4 and 5 kHz for 27Al and 31P, respectively. 2D 27Al–31P CP/MAS NMR spectra were obtained by encoding the aluminum evolution frequencies in an initial time period after the 90° pulse. Pure absorption phase NMR line shapes in both dimensions were obtained by cycling the phase of the 90° pulse using the TPPI scheme [21,22]. A more homogeneous polarization transfer was achieved by linear amplitude modulation of the spin-lock field on aluminum (the amplitude was decreased by 10% from 4 to 3.6 kHz). The contact time was 1.5 ms and the recycle delay was 300 ms. In a typical experiment, 2048 scans were accumulated for each of the 64 time-domain data points acquired in the aluminum dimension.
3 Results and discussion
The X-ray powder diffraction pattern of the solid matches exactly that of AlPO4-17 prepared with piperidine as template (JCPDS 85-1197). The absence of extra-peaks and the high intensity of the reflections indicate that the phase is pure and highly crystalline. The organic molecule is incorporated intact in the channels, as evidenced by 13C NMR. Differences in chemical shift between the molecule in the channels of the aluminophosphate and in the solution are an indication that the latter is doubly protonated. As mentioned in Section 1, the distance between N atoms in the organic molecule is of prime importance for the crystallization of AlPO4-17. Heating gels with the same molar composition but containing shorter diamines like N,N,N′,N′-tetramethylethylenediamine or N,N,N′,N′-tetramethyl-1,3-diaminoprpane led to the crystallization of AlPO4-21. These two templates are fragmented during synthesis and the obtained fragments are incorporated into the aluminophosphate framework. The presence of methyl groups on the nitrogen atoms is also crucial. Using 1,6-hexanediamine as template yielded layered materials for crystallization temperatures between 120 and 200 °C.
The 27Al NMR spectrum of the aluminophosphate is composed of two signals at ca. 15 and 40 ppm (Fig. 1). These signals have been previously assigned to the eight five-coordinate and the 10 four-coordinate Al atoms of the unit cell, respectively [23]. On the basis of X-ray powder diffraction and 27Al NMR, the solid prepared with the diamine is thus strictly similar to the previously reported AlPO4-17. However, the 31P NMR spectrum is complex and shows several lines with chemical shifts between –15 and –35 ppm, characteristic of PO4 tetrahedra (Fig. 1). After deconvolution, the spectrum consists of seven lines with relative intensities 1:1:1:3:1:1:1, suggesting the presence of at least nine crystallographically non-equivalent P sites in the structure (Table 3). The presence of several 31P NMR lines is in contradiction with previous data recorded on a solid prepared with piperidine [9,23], which indicates that the crystal symmetry of the title material is probably locally reduced. The local reduction of the symmetry was confirmed by 27Al 2D-5Q MAS NMR (Fig. 2). This technique provides enhanced resolution in the indirect dimension as compared with ordinary MAS spectra since the second order quadrupolar broadening is removed and the chemical shift dispersion is amplified, as it would be in a field approximately five times more intense. Nine signals can be separated in the indirect dimension f1: 5 (signals 1–5) correspond to AlO4 tetrahedra and 4 (signals 6–9) to pentacoordinated AlO5 species. The AlO4:AlO5 ratio between tetra and pentacoordinated Al species is 5:4, in agreement with the previous structure determination [9].
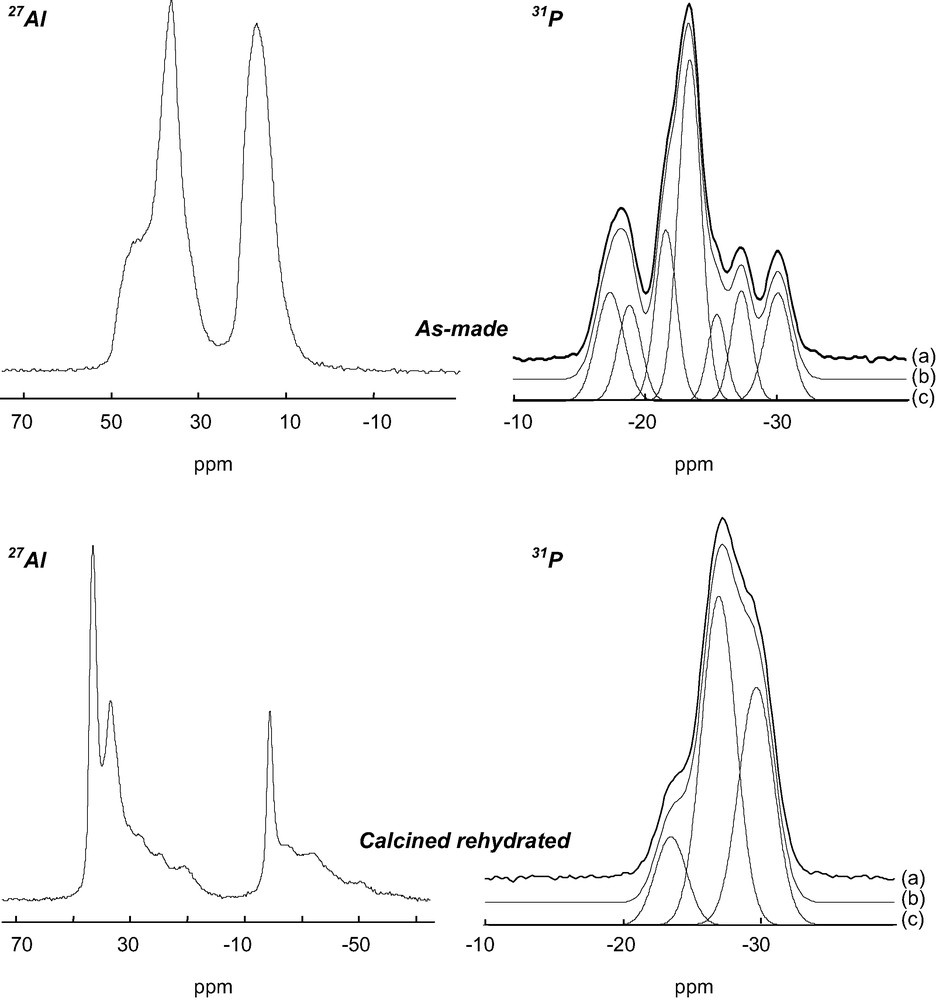
27Al and 31P NMR spectra of as made and calcined rehydrated AlPO-ERI. (a): experimental spectra, (b): simulated spectra and (c): decomposition of the simulated spectrum with Gaussian lines.
Chemical shifts (ppm) and relative intensity (%) of Gaussian components obtained after deconvolution of 31P NMR spectra of as-made and calcined rehydrated AlPO-ERI
As made | Calcined rehydrated | ||
δ (ppm) | Irel. (%) | δ (ppm) | Irel. (%) |
–17.34 | 10.98 | –23.79 | 11 |
–18.85 | 11.35 | –27.02 | 45 |
–21.65 | 12.29 | –29.91 | 44 |
–23.46 | 32.54 | ||
–25.52 | 10.21 | ||
–27.38 | 11.36 | ||
–30.8 | 11.27 |
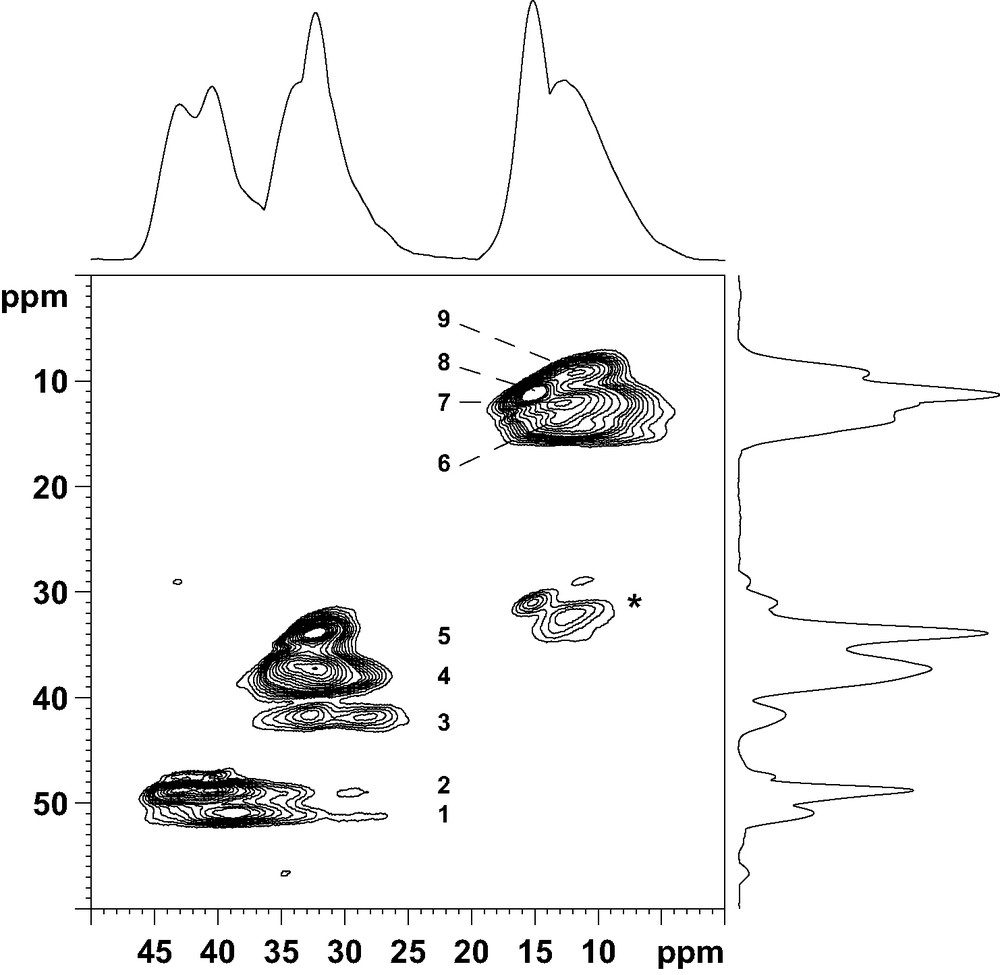
Sheared 27Al 2D-5Q MAS NMR spectrum of as-made AlPO-ERI. Asterisks denote spinning side bands.
The organic molecule is located inside the large ellipsoidal cages of the ERI structure (Fig. 3). Each ammonium group occupies approximately the same position as piperidine molecules in the structure reported by Pluth et al. [9], with a N–N distance of 6.82 Å. For piperidinium ions, C and N atoms in the rings could not be easily distinguished and the structure was refined in the hexagonal space group P63/m. By contrast, diamine molecules are ordered in the structure; all atom positions could be located and are fully occupied. The use of TMDAH molecules to synthesize AlPO4-17 materials results in a decrease of the symmetry of the aluminophosphate from hexagonal to monoclinic, as clearly evidenced by solid-state NMR. Therefore, this symmetry reduction seems to be induced by the templating molecule, but the exact mechanism is not clear except for the fact that the template is ordered in this space group. The lower symmetry leads to the existence of nine crystallographically non-equivalent P and Al sites in the unit cell, in agreement with 27Al and 31P NMR data. The positive charges of the organic molecule are balanced by extraframework OH groups, bridging Al atoms at the top and at the bottom of each cancrinite cage. Each diamine molecule is doubly protonated; so it has to be associated with two hydroxyl groups to maintain the neutrality of the framework. As a consequence, four of the six aluminum atoms located in the six-rings at the top and at the bottom of the cancrinite cage become pentacoordinated, the two others remaining tetrahedrally coordinated. From X-ray diffraction data, only one OH group (O1H) in the structure is a fully occupied position. The second one was considered to be statistically distributed over the two other positions in the cancrinite cage. In the case of a solid prepared with piperidine, OH groups can not be ordered (because of the hexagonal symmetry) and all the six Al atoms have to be considered as forming on average two thirds of the time a trigonal bipyramid and one third of the time a tetrahedron. The fact that one position is fully occupied in the solid containing the diamine may result from a specific interaction between the template and the framework. However, the ammonium head groups of the organic molecule cannot get in close contact with any of the extraframework OH groups because these groups are located in the cancrinite cage and are therefore not in the same cage as the template.
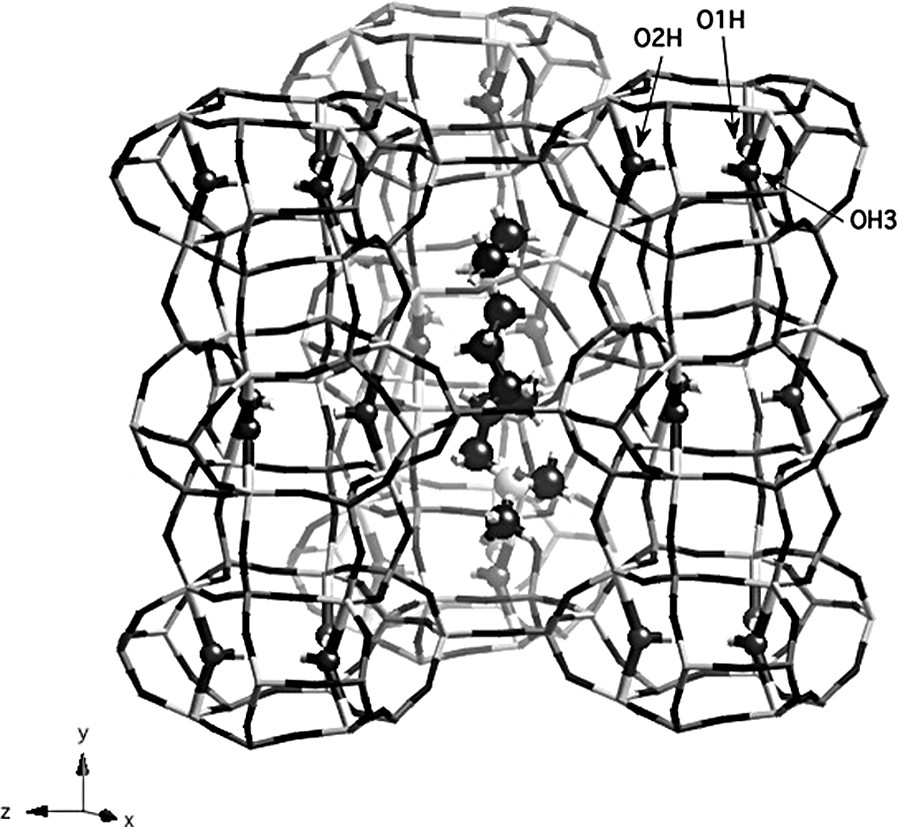
Structure of as-made AlPO-ERI showing the template in the large cage and OH groups in the CAN cage. O1H, O2H and O3H are labeled. Dark gray: P tetrahedra, light gray: Al polyhedra.
The presence of nine distinct signals in the 27Al 2D-5Q MAS NMR spectrum (Fig. 2) is a strong indication that the two OH groups are locally ordered. A possible arrangement for the extraframework hydroxyl groups in a cancrinite cage is represented in Fig. 4 (O1H and O2H are fully occupied, O3H is vacant. An equally valid arrangement would be to have O2H vacant and O3H fully occupied, and the following arguments could be made equally well with this assumption. Following this arrangement, Al(4), Al(6), Al(7) and Al(8) are pentacoordinated whilst Al(1), Al(2), Al(3), Al(5) and Al(9) possess a tetrahedral coordination. Al(5) and Al(9), located in the upper and lower six-rings of the cancrinite cage, respectively, are structurally different from Al(1), Al(2) and Al(3). Thus, in the tetrahedral region of the NMR spectrum (32–45 ppm) the two signals (signals 1 and 2) can be reasonably assigned to Al(5) and Al(9) and the group of three signals (signals 3–5) to Al(1), Al(2) and Al(3). Finally, the four signals around 15 ppm represent the five-coordinate Al(4), Al(6), Al(7) and Al(8) species. This assignment is supported by the following argument. The atoms Al(5) and Al(9) are tetrahedrally coordinated with Al–O–P bond angle values between 137.7 and 147.9° (Table 2), which indicates more or less undistorted environment (resonances at ca. 45 ppm). On the other hand, Al(1), Al(2) and Al(3) share 3 oxygens with P atoms in the same cancrinite cage, with Al–O–P bond angle values in the range of 146.0–152.1°, but the fourth oxygen connects aluminum atoms with P atoms located in a neighboring column, with a Al–O–P bond angle value higher than 170° (Table 2). This high value modifies the geometry and the distribution of charges around Al atoms, which probably influences their NMR chemical shift and line shape and reduces it to ca. 35 ppm (signals 3–5 in Fig. 2).
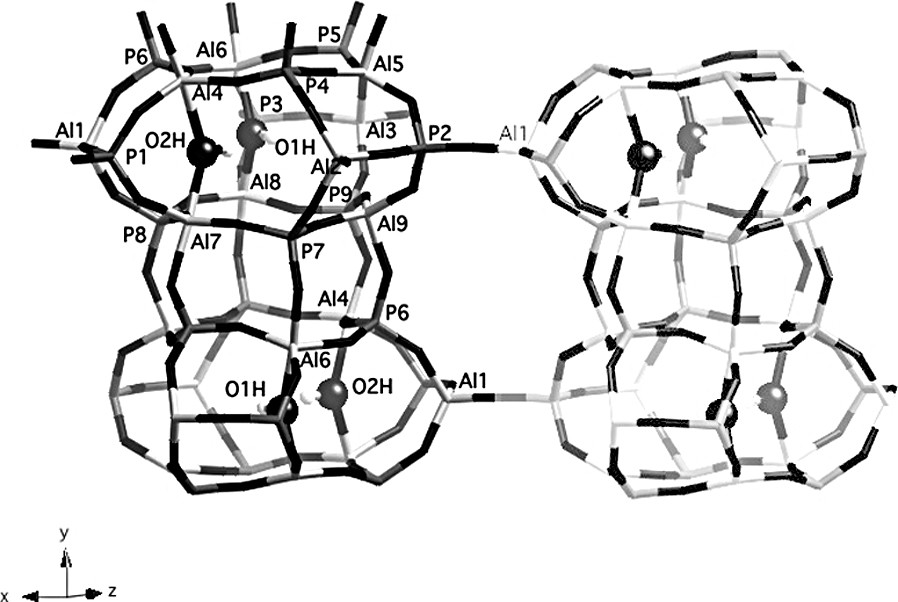
View of 2 cancrinite cages in the same column and a possible arrangement of OH groups (O1H and O2H fully occupied; O3H vacant). Al, P and extraframework OH groups are labeled. Dark gray: P tetrahedra, light gray: Al polyhedra.
More information about the local ordering of the framework was obtained from the 2D 27Al–31P CP/MAS NMR spectrum (Fig. 5). This technique, based on a polarization transfer between neighboring P and Al atoms, give a direct picture of framework connectivities. In the f1 dimension, representing the aluminum spectrum, the resolution is not as good as that of the 2D-5Q spectrum. However, the two types of tetrahedrally coordinated Al species, resonating at 43 and 35 ppm, are well separated. The correlation spectrum shows that the 2 P atoms with a chemical shift around –18 ppm are not bonded to aluminum atoms resonating at 43 ppm. Also, the P atom corresponding to the 31P NMR signal at –32 ppm is not connected to pentacoordinated Al species. From the representation of the structure given in Fig. 4, it can be seen that P(2) is not connected to five-coordinate Al species, since the Al(1) atom in the neighboring column is always a tetrahedral Al species. Thus, P(2) can be unambiguously associated with the 31P NMR signal at –32 ppm. P(1) and P(3) are connected to the five-coordinate Al species, located in the six-rings at the top and bottom of the cage, but not to the four-coordinate Al(5) and Al(9) resonating at 43 ppm. Therefore, they correspond to the two 31P NMR signals around –18 ppm. The 2D 27Al-31P CP/MAS spectrum indicates that all other phosphorus atoms are connected to both types of AlO4 tetrahedra. In the lower cancrinite cage, P(6) is bonded to 2 pentacoordinated Al(4) and Al(6) species, to Al(1) in the same cage and to Al(9) in the cage above (Fig. 4). From the 2D NMR spectrum, Al(1) and Al(9) must be tetrahedrally coordinated, which means that the O3H position is also vacant in the upper cage. These arguments can be continued down the column. This important result demonstrates that OH groups are ordered along a column (hexagonal prisms alternating with cancrinite cages) of the structure. However, the order is not maintained between the columns and the structure does not possess a long-range ordering.
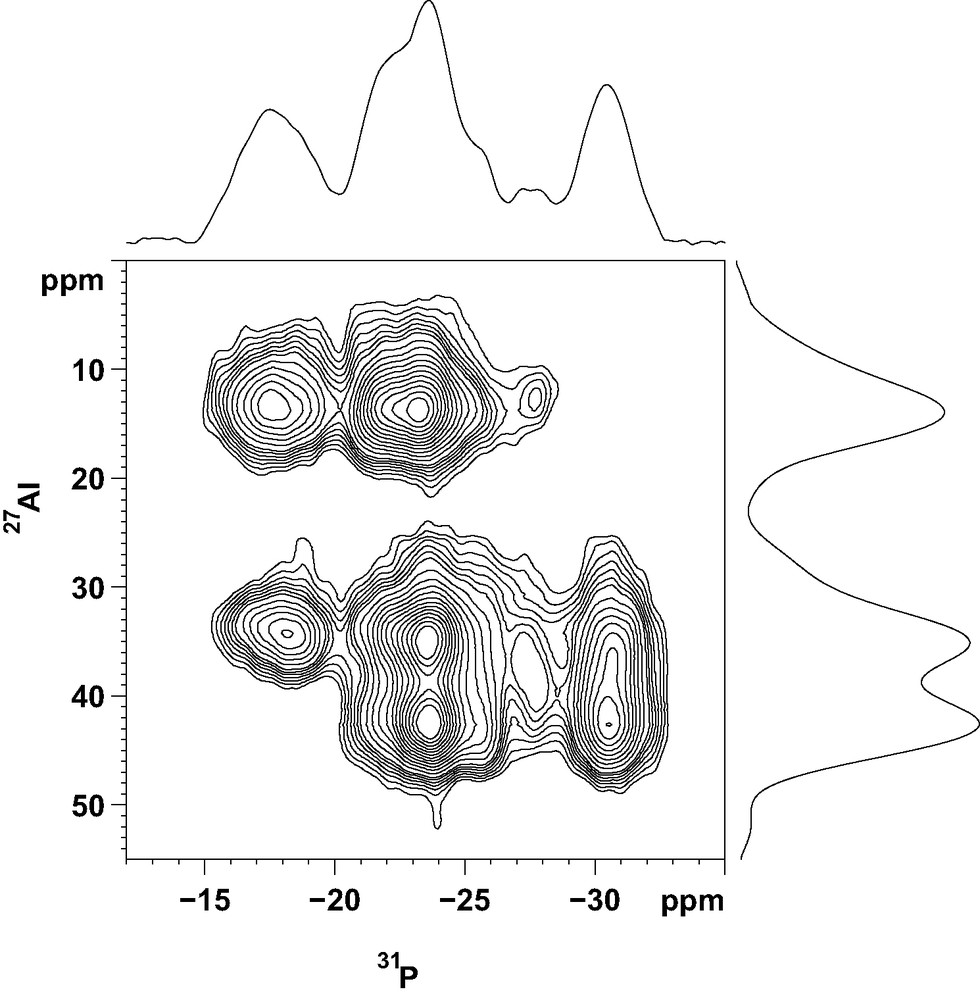
Two-dimensional 27Al–31P CP/MAS NMR spectrum of the as-made AlPO-ERI compound.
After calcination, 31P and 27Al NMR spectra of the solid (not shown) are composed of a unique signal at –30 and 35 ppm, respectively. In particular, the 27Al NMR signal indicates that all aluminum atoms are tetrahedrally coordinated and that extraframework OH groups have been removed. Both 31P and 27Al NMR spectra are modified upon rehydration of the calcined solid. The 27Al spectrum is complex and contains many lines between +45 and –50 ppm (Fig. 1). The signals ranging from –15 to –50 ppm can be unambiguously assigned to octahedrally coordinated Al species. They result from the modification of the coordination of framework Al atoms in the presence of water. The intensity of the signal represents approximately one third of the total intensity. The other signals between 5 and 45 ppm correspond to Al tetrahedra. Indeed, the 2D-5Q MAS NMR spectrum (not shown) shows that all aluminum atoms in this region possess an isotropic chemical shift characteristic of tetrahedrally coordinated species. However, some of these species possess very strong quadrupolar coupling constants, probably due to their interaction with non-framework water molecules. The 31P NMR spectrum of the rehydrated compound shows a major line at –27 ppm along with shoulders at –23.5 and –30 ppm (Fig. 1). It can be deconvoluted with three Gaussian lines with relative intensities 1:4:4 (Table 3), suggesting the existence of nine crystallographically non-equivalent P sites in the framework.
The X-ray powder diffraction pattern of the calcined rehydrated aluminophosphate is not very different from that of the as-made solid. This suggests that calcination and subsequent rehydration do not modify the topology of the framework, as it has been observed for other aluminophosphates. Moreover, the hydration process is reversible and both the X-ray powder pattern and NMR spectra of the dehydrated solid are restored upon evacuation at 100 °C. A TG experiment has shown that all water molecules are expelled from the channels at a temperature of ca. 100 °C, with a weight loss corresponding to 21.85 wt.%.
The unit cell of the calcined rehydrated solid is doubled along c but the symmetry is now P2(1)/n (Table 1), which again leads to nine crystallographically non-equivalent P and Al positions, in agreement with NMR data. Three Al atoms are octahedrally coordinated, also in agreement with 27Al NMR data. These atoms, namely Al(1), Al(2) and Al(3), are the atoms that were tetrahedrally coordinated in the as-made material (Fig. 6). By contrast, all Al atoms in the six-rings at the top and at the bottom of the cancrinite cage remain four-coordinated. The attached water molecules (O1W to O6W) point into the large ellipsoidal cage and not in the cancrinite cage, as extraframework OH groups did in the as made compound. One explanation for this could be that the water molecules could not easily enter the cancrinite cages because the 6MR that are accessible from the large cage are puckered. These six water positions are fully occupied and the three octahedrally coordinated Al atoms are topologically identical and related by a threefold axis. Despite this, the symmetry of the crystal is monoclinic but only slightly deviates from the hexagonal symmetry. The calcined rehydrated aluminophosphate also contains non-framework water molecules, i.e. molecules that are not directly bonded to framework aluminum atoms. There are 11 additional water molecules per unit cell, and all are located in the large ellipsoidal cage. They are distributed over 14 crystallographic positions, six positions being only half occupied. As mentioned above, cancrinite cages as well as D6R units do not contain any water molecules. The chemical formula of the calcined rehydrated solid is [Al9P9O36(H2O)6]·11 H2O, which corresponds to 21.8 wt.% H2O, in excellent agreement with the value obtained by thermal analysis.
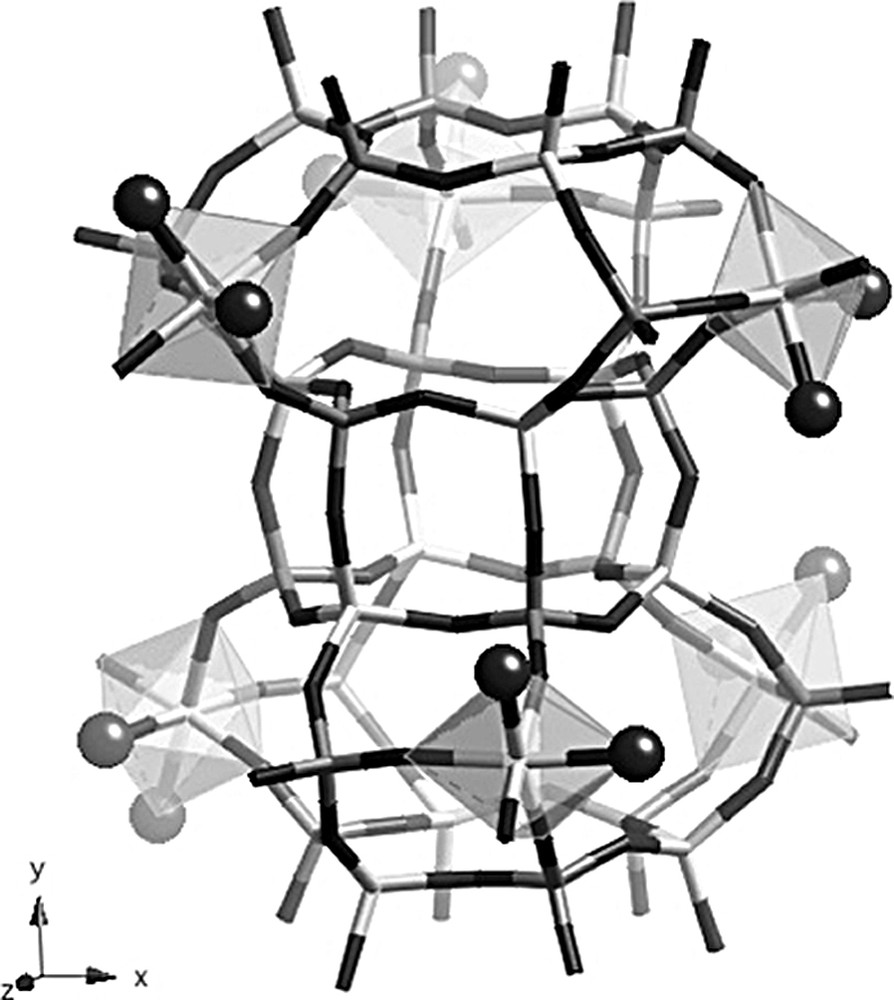
Structure of the calcined rehydrated solid showing the Al octahedra in the CAN cages. Dark gray: P tetrahedra, light gray: Al polyhedra. Dark spheres represent framework water molecules.
4 Supplementary material available
The crystallographic data (excluding structure factors) for the two structures have been deposited with the Cambridge Crystallographic Data Center for the as-made structure (CCDC 238862) and the Fachinformationzentrum Karlsruhe for the calcined rehydrated structure (CSD-Nr. 414026). Copies of the data can be obtained, free of charge, on application to CCDC, 12 Union Road, Cambridge CB2 1EZ, UK (e-mail: deposit@ccdc.cam.ac.uk), or Fachinformationzentrum Karlsruhe, Abt. PROKA, 76344 Eggen-Stein-Leopoldshafen, Germany (e-mail: crysdata@fiz-karlsruhe.de).