1 Introduction
The main target in recent solar-cell studies lies in lowering the fabrication cost without reducing the conversion efficiency. Much attention has thus been paid to thin-film solar cells [1,2], fabricated with inexpensive thin-film semiconductor materials such as amorphous silicon (Si), polycrystalline Si, and dye-sensitized TiO2. However, this type of solar cells has faced a serious deadlock that the use of expensive transparent conductive oxide (TCO) such as indium tin oxide is inevitably necessary for efficient current collection. In addition, the TCO has no enough electrical conductivity and thus we are compelled to adopt an elaborate solar-cell structure of series connection of a large number of tiny solar cells to avoid an increase in the ohmic loss [2], which leads to a significant cost increase. Furthermore, this series connection induces another cost increase because high-quality fabrication of tiny solar cells is required, for in this structure damage of one tiny cell ruins all the performance of tiny cells connected in series with it.
Solar to chemical conversion by use of a semiconductor/solution junction has attracted considerable attention as another new technology for solar energy conversion since the pioneering report of water photo-splitting with n-TiO2 in 1972 by Fujishima and Honda [3]. This method has great advantages in cost lowering over the thin-film solar cells, in that it does not need to use any expensive TCO and is easily adaptable to inexpensive thin-film semiconductor materials. The main difficulty in this method is that most of semiconductors with band-gaps suitable for solar energy conversion are chemically unstable in aqueous electrolytes [4,5]. Much effort has been done to overcome the difficulty, and several interesting solar to chemical conversion systems with considerably high efficiencies have been reported such as photodecomposition of hydrogen iodide (HI) with a Rh-loaded p-InP photocathode [6] or an n-WS2 photoanode [7], and photodecomposition of HBr and HI with a combination of a Rh-loaded p-InP photocathode and an n-WSe2 or n-MoSe2 photoanode [8]. Photodecomposition of water or other energy-storing reactions are also successfully achieved with stacked multilayer semiconductor electrodes [9–14].
Silicon (Si) is one of the most important semiconductors for solar energy conversion in view of an appropriate band-gap of 1.1 eV, abundance in natural resources, and non-toxicity, but easily oxidized and passivated in aqueous electrolytes. We reported fairly long ago [15–17] that single crystal n-Si electrodes loaded with metal nano-particles, immersed in a Br−/Br2 redox electrolyte, generated very high open-circuit photovoltages (Voc) of 0.62–0.64 V, considerably higher than those of conventional solid-state p–n junction Si solar cells of a similar simple structure. This finding provided a new way to utilize Si for solar to chemical conversion [18], but unfortunately the n-Si electrode showed no enough stability for long-term operation. Theoretically, it was expected [16,17] that the Si surface was stabilized by metal particles at metal-coated parts and passivating Si oxide layers at naked parts, but actually gradual Si oxidation occurred even beneath the metal particles, which led to the electrode degradation.
Recently, a number of studies have been reported on modification of Si surfaces with organic alkyl groups [19–26]. A large merit of surface alkylation is the improvement of Si stability against the surface oxidation in air [27] and in an aqueous redox electrolyte [28], without any increase in the surface carrier recombination rate. However, it is also reported [29,30] that the surface alkylation tends to retard interfacial electron transfer at the Si/redox electrolyte contacts. We reported in a previous paper [31] that this dilemma could be overcome by metal nano-dot coating because the metal nano-dots acted as an effective catalyst for interfacial electron transfer reactions.
The above finding is of great importance because it finally provides an effective way to utilize Si for solar to chemical conversion. In the previous work [31], we studied the behavior of the methylated and Pt nano-dotted n-Si electrode in 8.6 M HBr + 0.05 M Br2 with a highly positive redox potential to get high Voc′s. In the present work, we have studied the behavior of the methylated and Pt nano-dotted n-Si in 7.6 M HI (or 7.6 M HI + 0.05 M I2) to examine a possibility of efficient solar to chemical conversion via photodecomposition of HI into H2 and I2 (or I3–) with no external bias.
2 Experimental
Single crystal n-Si (111) wafers of the resistivity of 1 ~ 5 Ω cm and the thickness of 825 ± 25 μm, donated by New Win Go Co. Ltd., were used. The Si surfaces were cleaned by immersing in a boiling mixture of conc. H2SO4 and 30% H2O2 (3:1 in volume) for 15 min and in 5% HF for 5 min. The hydrogen (H)-terminated Si surfaces were obtained by further immersion in a boiling mixture of 25% aqueous NH3, 30% H2O2, and water (1:1:5 in volume) for 15 min, followed by consecutive immersion in 5% HF for 5 min and 40% NH4F for 15 min.
The methylation of the Si surface was, in the present work, obtained by a method of photochlorination followed by Grignard reaction, which was reported by Bansal et al. [21] and Okubo et al. [25]. Namely, an H-terminated n-Si (111) wafer was put in a saturated chlorobenzene solution of phosphorus pentachloride (PCl5) and illuminated at 100 °C for 1 h. The wafer was then put in a saturated diethyl ether solution of CH3Li and kept for 2 h at room temperature. All the procedures were done under a dried argon atmosphere by use of the schlenk technique. The Si wafer thus methylated was finally washed with diethyl ether, ethanol and pure water.
The n-Si wafer was attached to a photoelectrochemical cell with an O-ring (effective area: 0.18 cm2) in a form of a ‘window’. Ohmic contact with n-Si was obtained with indium-gallium alloy. A platinum plate was used as the counter electrode, and an Ag|AgCl (sat. KCl) electrode was used as the reference electrode. Platinum nano-particles were deposited electrochemically on the methylated Si surface at −1.0 V vs. Ag|AgCl in 5 mM K2PtCl6 + 100 mM LiClO4. The amount of electricity passing across the Si surface was 83 mC cm−2. All chemicals were of reagent grade and used without further purification. Pure water with a resistivity of 18 MΩcm was obtained by a Milli-Q purification system.
Photocurrent density (j) vs. potential (U) was measured with a ‘Hokuto-Denko HSV-100 standard voltammetry tool’, composed of a combination of potentiostat, potential programmer, and recorder. The electricity passing across the electrode was measured with a digital coulomb meter (Nikko-Keisoku NDCM-3). Surface inspection was carried out with a Hitachi S-5000 high-resolution scanning electron microscope (SEM). X-ray photoelectron spectroscopic (XPS) analysis was performed with a Shimadzu ESCA-1000 spectrometer using an Mg Kα line.
3 Results and discussion
The methylation of the n-Si surface by the above-described chemical treatment was confirmed by XPS analysis, as reported previously [31]. The methylated (CH3-terminated) Si surface gave the carbon-1s XPS peak at 283.8 eV that was attributed to carbon in surface Si–CH3 bonds, in contrast to non-methylated (H-terminated) Si surfaces. This assignment was in good agreement with the work reported by other researchers [25,32]. A very rough estimation of the surface coverage (θ) of CH3 group from the C and Si XPS peaks, using the method of Himpsel et al. [33], gave a value of around 70%, which was somewhat higher than a reported value of less than 50% [24] probably owing to an influence of contaminating organic compounds at the Si surface.
Fig. 1 shows scanning electron micrographs (SEMs) of (A) CH3-terminated and Pt-dotted and (B) H-terminated and Pt-dotted n-Si surfaces just after Pt deposition. Circular Pt particles were deposited fairly homogeneously all over the n-Si surface in both cases, though the size of the Pt particles for the CH3-terminated and Pt-dotted n-Si was much larger, and the density of them was much less, than the corresponding values for the H-terminated and Pt-dotted n-Si. The current density vs. potential for electrodeposition of Pt on the CH3-terminated n-Si was similar to that on the H-terminated n-Si, strongly suggesting that Pt for the CH3-terminated n-Si was deposited on non-methylated (uncovered) parts of the Si surface, not on the methyl group of the methylated parts. It will be reasonable to assume that the methylated Si surface with the coverage of about 50% has non-methylated parts (domains) of nano-sizes here and there, at which Pt can be deposited.

SEMs of (A) CH3-terminated and Pt-dotted and (B) H-terminated and Pt-dotted n-Si surfaces just after Pt deposition.
Fig. 2 shows the photocurrent density (j) vs. potential (U) for (A) CH3-terminated and Pt-dotted and (B) H-terminated and Pt-dotted n-Si (111) electrodes in 7.6 M HI + 0.05 M I2 under simulated solar (AM 1.5 G, 100 mW cm–2) illumination. Solid curves were observed at the initial stage, whereas dotted curves were observed after 24-h illumination. The CH3-terminated and Pt-dotted n-Si electrode gave an efficient j–U curve, yielding a much higher open-circuit photovoltage (Voc) than the H-terminated and Pt-dotted n-Si electrode. It is to be emphasized that it is for the first time that such an efficient j–U curve as Fig. 2A was obtained for n-Si in a redox electrolyte of 7.6 M HI + 0.05 M I2 with a fairly less positive redox potential. This clearly indicates the effectiveness of Pt nano-contact and surface methylation. It is to be noted also that the j–U curve for the CH3-terminated and Pt-dotted n-Si electrode was quite stable, showing almost no change during 24-h illumination, contrary to the case of the H-terminated and Pt-dotted n-Si electrode. This also indicates the effectiveness of the Pt nano-contact and surface methylation. Methylated but no Pt-dotted n-Si electrodes showed only poor j–U curves, with the photocurrent starting at a fairly positive potential and increasing very gradually with the potential, though the j–U curves were stable for more than 30 min under continuous illumination, as already reported [31].

j vs. U curves for (A) CH3-terminated and Pt-dotted and (B) H-terminated and Pt-dotted n-Si (111) electrodes in 7.6 M HI + 0.05 M I2 under simulated solar (AM 1.5 G, 100 mW cm–2) illumination. Solid curves were observed at the initial stage, whereas dotted curves were observed after 24-h illumination.
Fig. 3 shows Si-2p XPS peaks for (A) CH3-terminated and Pt-dotted and (B) H-terminated and Pt-dotted n-Si surfaces. Similarly to Fig. 2, solid curves were observed at the initial stage just after the Pt deposition, whereas dotted curves were observed after the 24-h illumination. The H-terminated and Pt-dotted n-Si showed an additional high-energy peak at 103.5 eV, attributed to formation of SiO2 (Fig. 3B), suggesting that the H-terminated n-Si(111) surface was slightly oxidized during the Pt deposition. The oxidation will occur at naked (non-Pt-deposited) parts of the Si surface, as reported [16,17]. The oxidation was prevented by the methylation (Fig. 3A), most probably because the surface methyl group formed a hydrophobic thin layer on the Si surface and prevented the approach of water molecules to it. It is likely that the positive shift of the onset potential of the photocurrent (or a decrease in the Voc) for the H-terminated and Pt-dotted n-Si electrode in Fig. 2 is caused by the surface oxidation mentioned above, because the surface oxidation induces a positive shift in the flat-band potential (Ufb) of n-Si owing to the formation of an electrical double layer of surface dipoles (Siδ+−Oδ−).
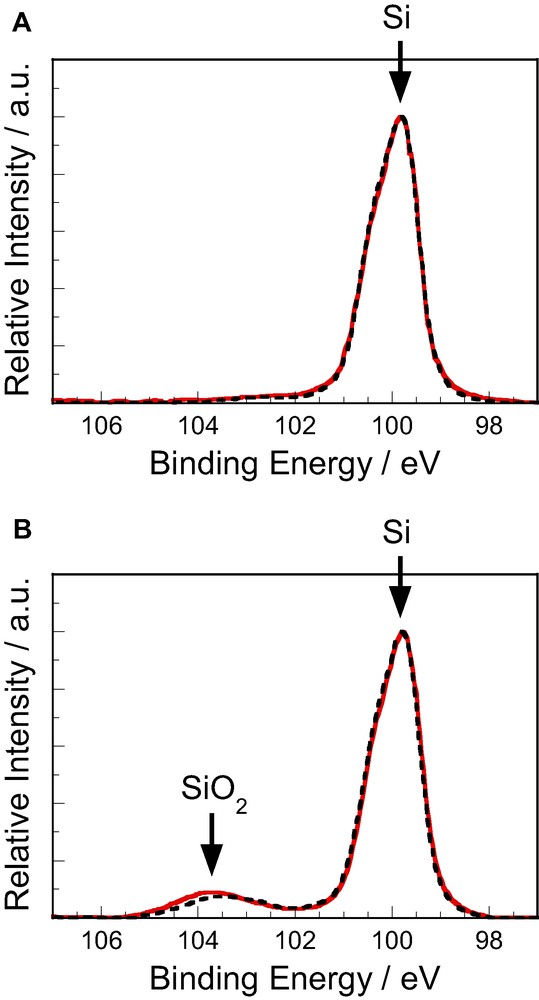
Si-2p XPS peaks for (A) CH3-terminated and Pt-dotted and (B) H-terminated and Pt-dotted n-Si surfaces just after the Pt deposition. Solid curves were observed at the initial stage, whereas dotted curves were observed after 24-h illumination.
One may note that almost no further oxidation of the Si surface proceeded during the illumination in the (HI + I2) solution for both the electrodes (Figs. 3A and B) whereas the j–U curve for the H-terminated and Pt-dotted n-Si electrode degraded severely after the 24-h illumination (Fig. 2B). The degradation can be attributed to gradual progress of the Si oxidation just beneath the Pt nano-particles, which leads to formation of a thin insulating layer between Si and Pt, responsible for the degradation. Almost no change in the height of the Si-2p peak attributed to Si oxide for the H-terminated and Pt-dotted n-Si electrode can be understood if we take into account that only a very small part of the whole Si surface is covered with Pt nano-particles and thus an increase in the amount of the Si oxide by the above oxidation is negligibly small.
A success of development of a new-type Si electrode with metal nano-contact and surface methylation has enabled us to accomplish efficient and stable solar to chemical conversion through photodecomposition of HI into H2 and I2 with no external bias. Experiments were done with a one-compartment cell, such as shown schematically in Fig. 4, for convenience. The photocurrent was measured using a fresh electrolyte at every experiment in a photo-stationary state before the oxidized product (I2 or I3−) on the n-Si electrode diffused to the counter Pt electrode and affected the hydrogen evolution reaction on it.
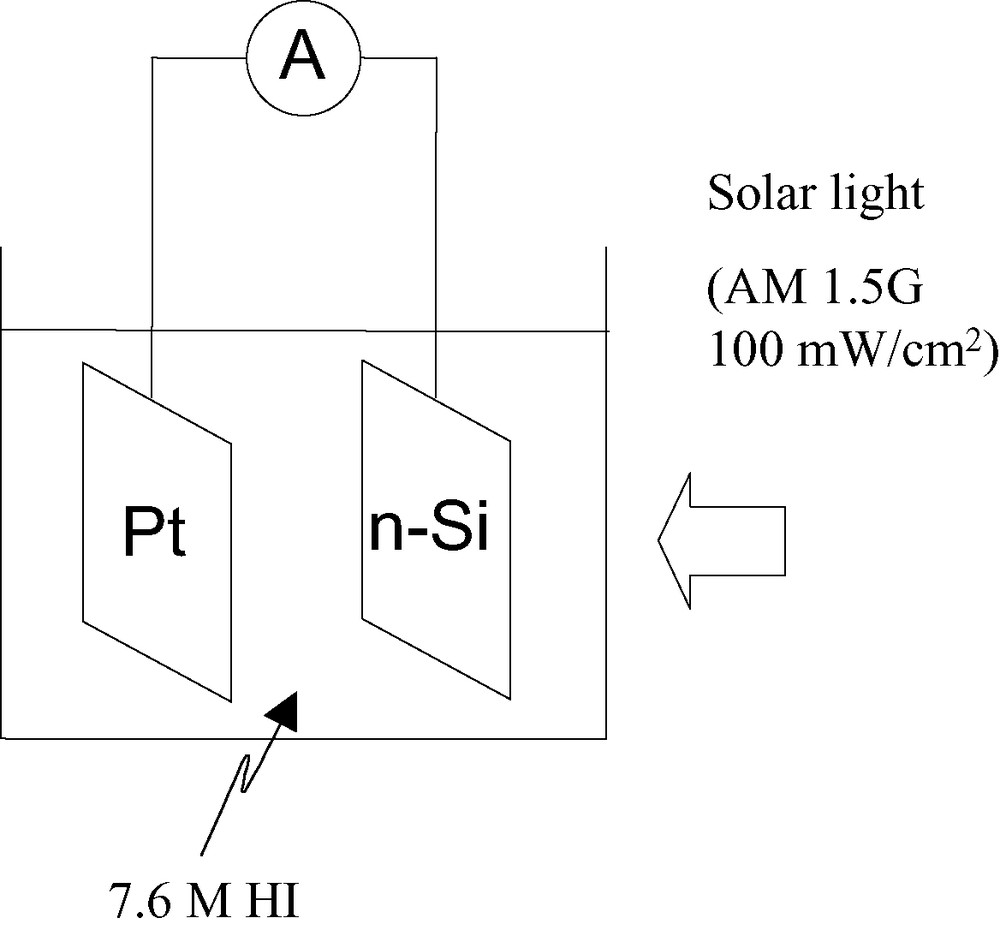
Schematic illustration of a photoelectrochemical cell for efficient solar decomposition of hydrogen iodide (HI) into hydrogen (H2) and iodine (I2 or I3–).
The solar to chemical conversion efficiency
(1) |
(2) |
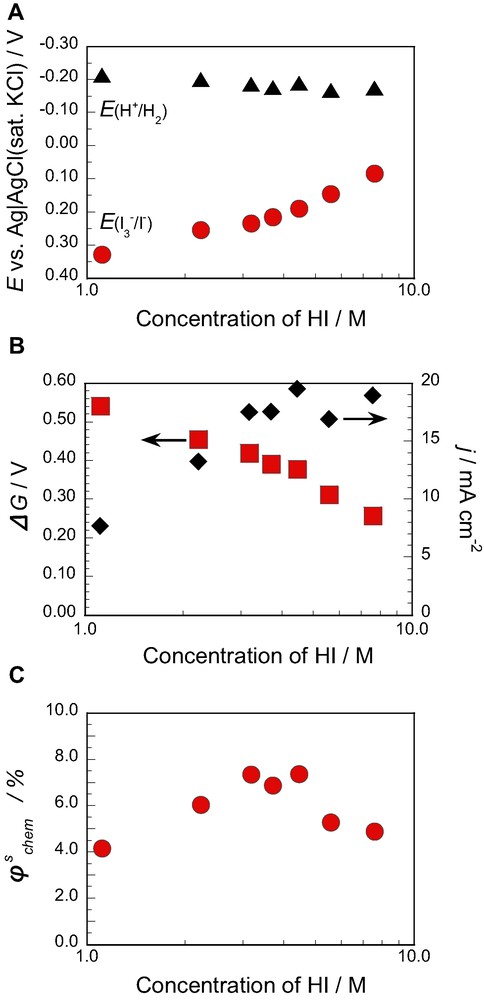
(A) The redox potentials for oxidation of I− to I2 (or I3–), E(I3−/I−), and hydrogen evolution, E(H+/H2), (B) the Gibbs energy ΔG for the decomposition of HI into H2 and I2 (or I3–) and the observed photocurrent density (j), and (C) the solar to chemical conversion efficiency
The
Fig. 6 shows the j–U curve for the photo-induced oxidation of I− ions to iodine (I2 or I3–) on the CH3-terminated and Pt-dotted n-Si (111) electrode, compared with that for the hydrogen evolution on a Pt-plate electrode in the same electrolyte solution. The anodic current for the n-Si electrode and the cathodic current for a Pt electrode became equal in the absolute value at about −0.32 V, which explained the principle of operation of the solar decomposition cell of Fig. 4. The conversion efficiency obtained in the present work is high enough, as mentioned above, but this value can still be increased by optimization of the experimental parameters such as the coverage of the CH3 group and the quantity and the size of the Pt particles. The matt-texture treatment of the n-Si surface to decrease the light reflectivity at the surface will also be important in increasing the photocurrent density.

The j–U curves for the photo-oxidation of I− on n-Si (111) and hydrogen evolution on Pt, in order to explain the principle of operation of the solar decomposition cell of Fig. 4.
In conclusion, the present work has revealed that an n-Si electrode with metal nano-contact and surface methylation gives a high Voc even in a redox electrolyte of 7.6 M HI (or 7.6 M HI + 0.05 M I2) and thus can be used for efficient solar to chemical conversion through photodecomposition of HI into H2 and I2. The solar to chemical conversion efficiency
Acknowledgements
The authors thank Prof. K. Mashima and his research group in Osaka University for their kind instruction and assistance in experiments on surface methylation of Si.