1 Introduction
Purification of water from organic contaminants, a major challenge of 21st century, can be performed through photocatalytic oxidation of the contaminants, by exposing a suspension of TiO2 particles to UV radiation. However, separation of the particles from the purified water is a problem. An alternate method consists in using a short-circuited photoelectrochemical cell [1]. The organic contaminants are oxidised at the oxide photoanode (typically TiO2), and dissolved dioxygen is reduced at the cathode. In that case, the efficiency is dramatically affected by kinetic limitations at the electrodes, and is improved only if an extra potential is added in the circuit. Nakato et al. have proposed to incorporate silicon into a TiO2 photoanode in order to benefit from an extra photovoltage [2]. However, a simpler way to obtain an extra potential is by using a photoactive cathode. An inexpensive method, scalable to large-area, would be elaborating such a photocathode from a thin-layer p-type semiconductor, such as amorphous hydrogenated silicon (a-Si:H). In a solar-energy powered purification reactor, the oxide photoanode makes use of the high-energy photons only, so that the low-energy photons are still available for the a-Si:H photocathode, thereby supplying a photopotential for free. Ideally, no extra potential would be necessary, and the cell could operate just by short-circuiting the two electrodes. The scheme of such a purification cell is shown in Fig. 1.
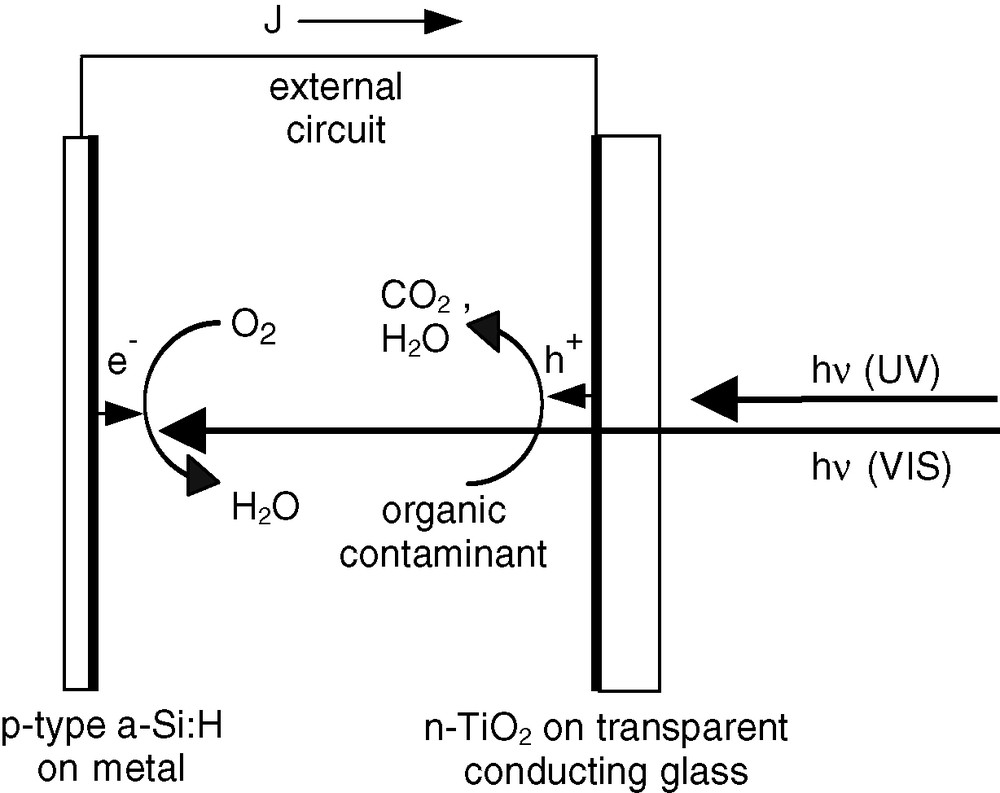
Scheme of a photoelectrochemical water purification cell with two active photoelectrodes and no external potential.
In order to design such a photocathode and optimise its properties, we have undertaken a fundamental study of bulk crystalline silicon and amorphous hydrogenated silicon photocathodes for the reduction of dioxygen.
2 Experimental
Single-crystal (100)-oriented p-Si electrodes were elaborated from p-type wafers supplied by ITME (Poland). Ohmic back-contact was obtained by vacuum evaporation of an (Au + 1% Al) alloy on the heated wafer. Square pieces (5 × 5 mm2) were cut and machined as 5-mm diameter disks. Rotating-disk electrodes (RDE) were then obtained by gluing these disks with electrically conductive epoxy on a brass holder, and encapsulated with wax in a polytrifluorochloroethylene holder.
Thin-layer a-Si:H electrodes were made by plasma-assisted chemical vapour deposition (PECVD) from silane in an rf low-power plasma (total pressure 25 mTorr, rf frequency 13.56 MHz, rf power density 0.15 W cm–2, substrate temperature 250 °C, deposition rate 1 μm h–1). Doping was obtained by adding appropriate amounts of diborane (p-type doping) or phosphine (n-type doping) into the gas. Stainless-steel M3 screws with machined and polished heads were used as the substrates, providing appropriate geometry for RDE without any further machining. Ohmic back-contact to the stainless-steel substrate was obtained by starting the a-Si:H deposit with a highly doped p+ layer (1% diborane in silane). This preparation method is very versatile, and various structures were prepared (p+/p or p+/i/n+), as it will be described in due time.
In the course of this work, the surface of the crystalline p-Si electrodes and a-Si:H structures was modified by various treatments, that will be presented when needed.
The electrodes were characterised as RDE in electrolytes of various pHs [(0.1 M HF + 1 M NH4Cl + 1 M HCl), pH 0; 0.5 M H2SO4, pH 0.3; acidic phosphate buffer solution, pH 2; neutral phosphate buffer solution, pH 6.5; alkaline phosphate buffer solution, pH 12]. Since special attention was paid to the dioxygen reduction reaction, the electrolytes were bubbled either with pure nitrogen or with air. The cell consisted of the RDE, a Pt-wire counter electrode and a saturated calomel reference electrode. The working electrode could be illuminated with a 250 W tungsten-lamp fed with a variator. The photon flux was determined from the response of a calibrated silicon photodiode placed at the same position as the working electrode. In the following, the light intensity is given as the percentage of the maximum power, which corresponds to a photon flux of ~1017 s−1 cm−2 [3].
3 Bare p-Si electrodes
Bare p-Si photocathodes (either crystalline Si or a-Si:H) exhibit large overpotentials for hydrogen evolution and dioxygen reduction [3]. This is indicative of poor catalytic properties of the Si surface. It appears that cathodic prepolarisation in the hydrogen evolution region leads to improved catalytic properties, an effect probably associated with the formation of active sites upon breaking of the Si lattice by hydrogen insertion, but these improvements disappear when the potential is swept in the open-circuit potential region (“curing out” of the active sites, see large hysteresis in the voltammograms of Fig. 2). Furthermore, the silicon surface is sensitive to oxidation: any excursion of the potential above open-circuit potential results in dramatic changes of the voltammogram, associated with irreversible formation of an oxide layer. This phenomenon may be avoided by working in fluoride electrolyte, but in that case Si dissolution takes place, which is equally detrimental to electrode lifetime. These two problems (catalytic properties and stability) must be addressed for the realisation of useful photocathodes.
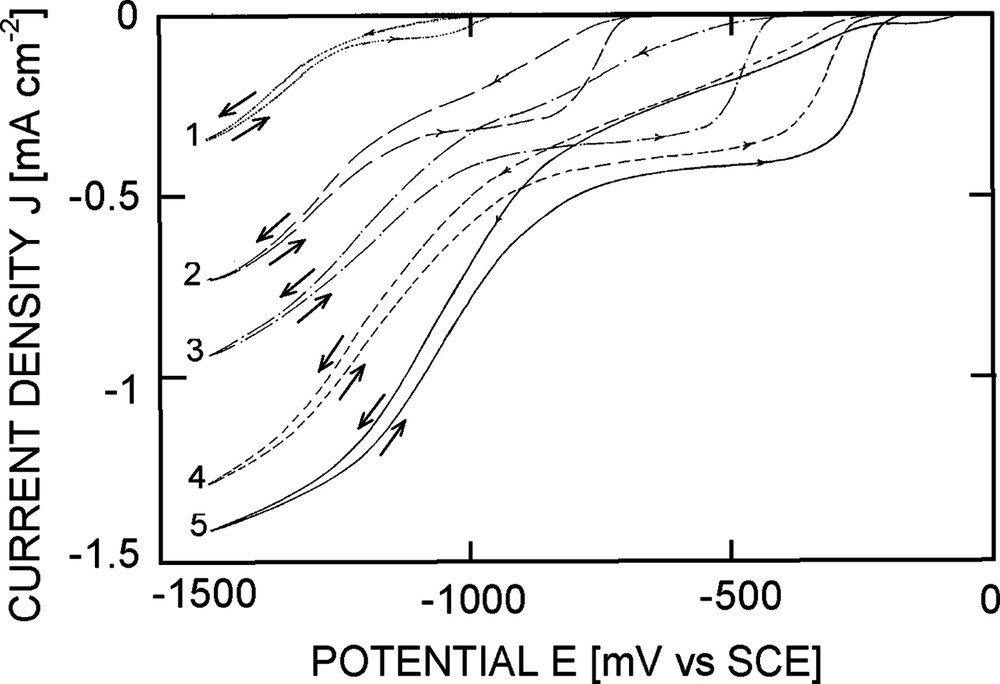
Cyclic voltammograms of p-Si (100) in different media (saturated with air by bubbling). Curve (1): alkaline phosphate; (2): neutral phosphate; (3): acidic phosphate; (4): (0.5 M H2SO4, pH 0.3); (5): (0.1 M HF + 1 M NH4Cl + 1 M HCl). Electrode rotation rate 900 rpm, potential scan rate 50 mV s−1, light intensity 17%. Note that the dioxygen reduction plateau is well defined only on the positive potential sweep (strong hysteresis), obtained after prepolarisation of the electrode for a few minutes at a negative potential.
4 Functionalised crystalline p-Si electrodes
Remedies to the problems of catalytic properties and electrode stability were sought through surface functionalisation.
4.1 Catalytic effect from Pt islet deposition
Deposition of platinum onto the surface of a p-Si (100) photocathode has long been known to lead to improvement of its electrocatalytic properties (i.e. improvement of its photoelectrochemical efficiency) for the hydrogen evolution reaction [4–6]. Here we have investigated the effect of Pt deposition with respect to the reduction of dissolved dioxygen.
The electrochemical platinisation of the p-Si (100) surface was carried out under potentiostatic conditions under illumination. The electrolyte consisted of (0.001 M H2PtCl6 + 0.10 M HCl) pH 1. A negative potential (nucleation pulse) was applied first, then deposition was pursued at a less negative potential. The potentials and durations of these two steps were varied and the resulting deposits examined by SEM. Some surfaces show bright Pt-rich areas, others show a small number of Pt islets, depending upon the operating conditions. As it is expected from general knowledge on electrodeposition [7], the density of islets appears determined by the potential and duration of the initial pulse, whereas their size is largely determined by the duration of the deposition step.
The voltammograms of platinised p-Si photocathodes exhibit a reduced hysteresis and a shift of the curves toward positive potentials, indicating improved kinetics of hydrogen evolution and dioxygen reduction as compared to bare p-Si photocathodes (see Fig. 3). However, for crystalline Si as well as for a-Si:H, the largest improvement observed for dioxygen reduction is still too small to compensate for the initially poor kinetics, that is, the current at a given potential seldom exceeds that at a bare Pt cathode [8].
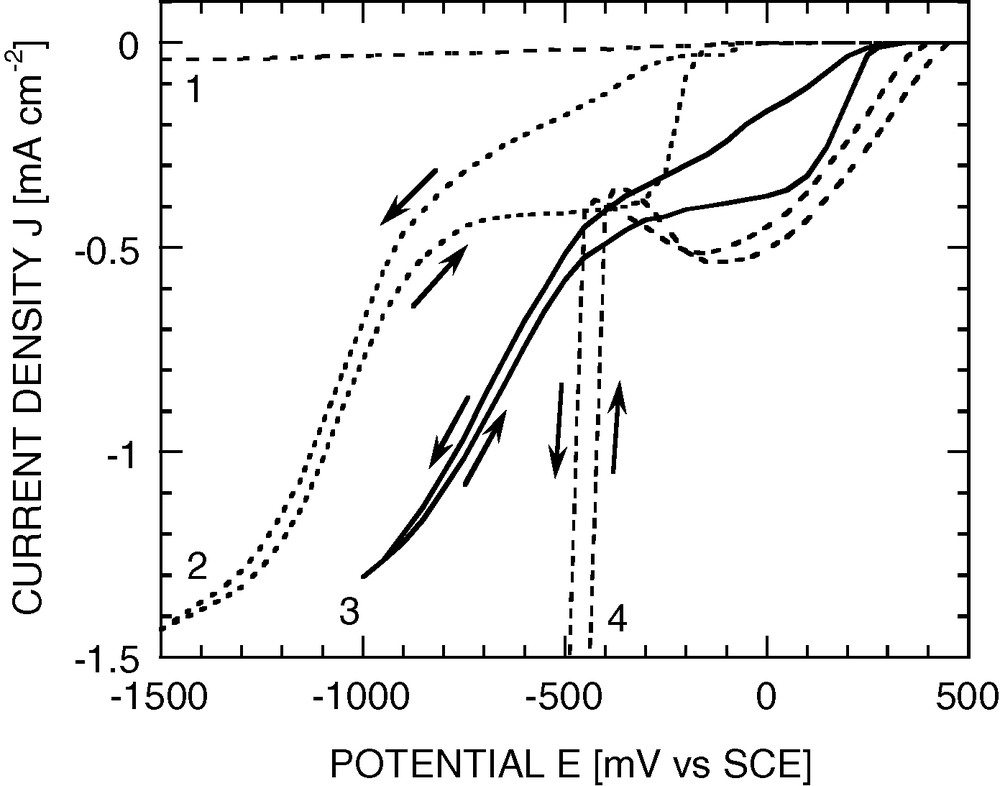
Effect of Pt deposition on a p-Si (100) photocathode in air-saturated 0.05 M H2SO4. RDE 900 rpm. Light intensity 17%. Sweep rate 50 mV s–1. (1) Bare or platinised Si in the dark; (2) bare Si under illumination; (3) typical p-Si electrode with electrodeposited Pt (electrolyte 0.10 M HCl + 0.001 M H2PtCl6, nucleation –3 V, 20 ms, deposition –0.02 V, 30 s), under illumination; (4) bulk Pt cathode.
4.2 Photoelectrode stabilisation
Photoelectrode stabilisation was addressed using various organic overlayers.
4.2.1 Anodic alkylation in a Grignard reagent
Anodic decomposition of a Grignard reagent RMgX (R = alkyl chain, X = I, Br or Cl) at a hydrogenated silicon electrode surface has been shown to lead to covalent grafting of organic species [9,10]:
≡SiH + 2RMgX + 2h+ → ≡SiR + RH + 2MgX+
The obtained surfaces have been characterised using infrared spectroscopy and X-ray photoelectron spectroscopy and the reaction mechanism has been studied in detail. An interesting aspect of the modified surface is the fact that the alkyl chains are covalently anchored to silicon, which is expected to bring significant chemical stabilisation.
Several wafers were modified with methyl and decyl groups. Typically, silicon was anodised in a 1 M solution of RMgX in diethylether, under constant 0.5 mA cm–2 current density during 5 min. Then 5-mm diameter RDEs were made from the modified wafers, and were characterised in the same electrolytes as above. The obtained methylated or decylated surfaces exhibit improved stability to oxidation as compared to the hydrogenated surface, when exposed either to alkaline medium or to oxidising medium (e.g., dilute solution of Fe3+ ions). The organic coating is not found to affect the voltammograms in a detrimental fashion. A slight shift of the hydrogen evolution toward positive potentials is even observed (see Fig. 4). In other words, the organic layer does not appear to hinder electrochemical transfer, and the catalytic properties of the surface even appear somewhat improved as compared to the bare Si surface.
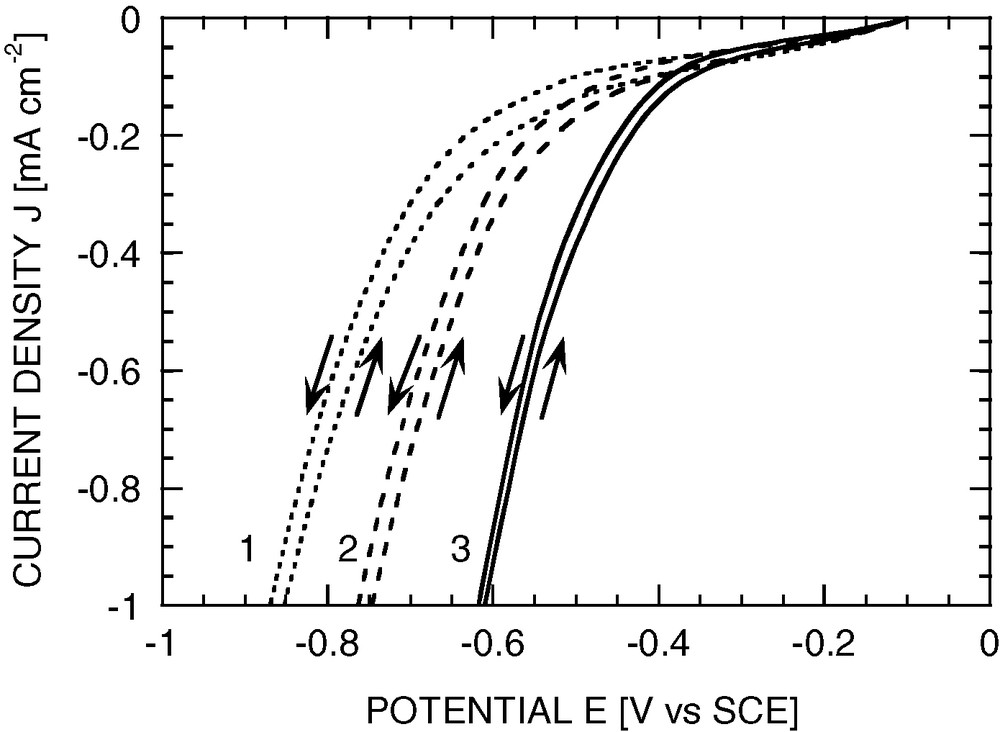
Typical voltammograms of p-Si photocathodes modified with organic groups. Electrolyte 1 M HF (pH 1); RDE 400 rpm; sweep rate 30 mV s–1. The dioxygen reduction current is lower than in Fig. 1, because here no negative prepolarisation was applied. (1) Bare p-Si electrode; (2) methylated; (3) decylated.
4.2.2 Anodic grafting of polymer films
When Grignard reagents formed from unsaturated groups (phenyl, chlorophenyl, vinyl, ethynyl) are used, most often the grafting reaction is not self-limited to a monolayer, but a polymeric layer is formed instead [11]. The polymers formed are covalently anchored to the silicon surface. In the case of phenyl and chlorophenyl precursors, it appears that the phenyl ring is preserved in the polymerisation reaction, which ends with a polyphenylene polymer. In the case of vinyl or ethynyl Grignards, addition reactions appear to play a major role, and a significant fraction of the multiple bonds are broken in the polymer formed.
Improved stability of silicon electrodes was obtained by the grafting of such polymer layers. This case appears of special interest as compared to that of alkyl groups, since the thickness of the polymer layer can be tuned by changing the conditions of the treatment. Thicker films, obtained by passing a larger coulometric charge, provide increased stability. Here again, a common feature of all these treatments is that the organic coating is covalently anchored to the silicon surface. The resulting photoelectrodes were tested in H2SO4 electrolyte. It was found that, up to thicknesses on the order of 5–10 nm, the organic layers do not bring a significant overpotential, and even provide some catalytic effect for hydrogen evolution, as compared to the initial hydrogenated p-Si electrode surface.
5 Functionalised p-type a-Si:H electrodes
The effect of platinum islets on the performance of amorphous hydrogenated (a-Si:H) photocathodes has been investigated, and sputtered Pt has also been tested in comparison with electrochemical deposition. Both methods lead to results similar to those obtained on crystalline p-Si.
The organic modification method has been shown to work on the a-Si:H surface, provided it is hydrogenated prior to the anodic treatment, by exposing it to HF vapour during a few seconds. As an alternate method for electrode stabilisation, inorganic layers of indium–tin oxide (ITO) and WO3–x have been prepared using d.c. magnetron sputtering. For the case of ITO, an ITO target was used in an argon plasma. The obtained films are sufficiently conducting that thicknesses on the order of 100 nm can be used without any problem of series resistance. For the case of WO3–x, a tungsten target was used in an argon plasma with small amounts of oxygen (reactive sputtering).
Finally, electrodes were submitted to the two treatments (in a first step, formation of an organic or inorganic protective layer, and Pt deposition in a second step), and their performance as photocathodes was optimised by varying the protective coating and the conditions of deposition of the Pt islets. The thickness of the protective layer has been optimised with respect to photoelectrode stabilisation and performance: the layer has to be thick enough to provide efficient stabilisation, but an exceedingly thick layer leads to an extra overpotential under operating conditions. It has been found that, for a polymer coating (e.g., polyphenylene obtained by anodisation of Si in phenylmagnesium bromide electrolyte), an optimum thickness is about 5 nm. Alternately, an ITO layer can be as thick as 50 nm without any problem of series resistance. Platinum deposition has been optimised too. It has been found that quasi-continuous Pt layers, as obtained by vacuum sputtering, and islet-deposits, as obtained by electrodeposition, lead to comparable overall performance, when the thickness is optimised. The best of these performances are just slightly better than those of a bare Pt cathode (maximum positive shift of the dioxygen reduction wave is about +0.1 V, see Fig. 5).
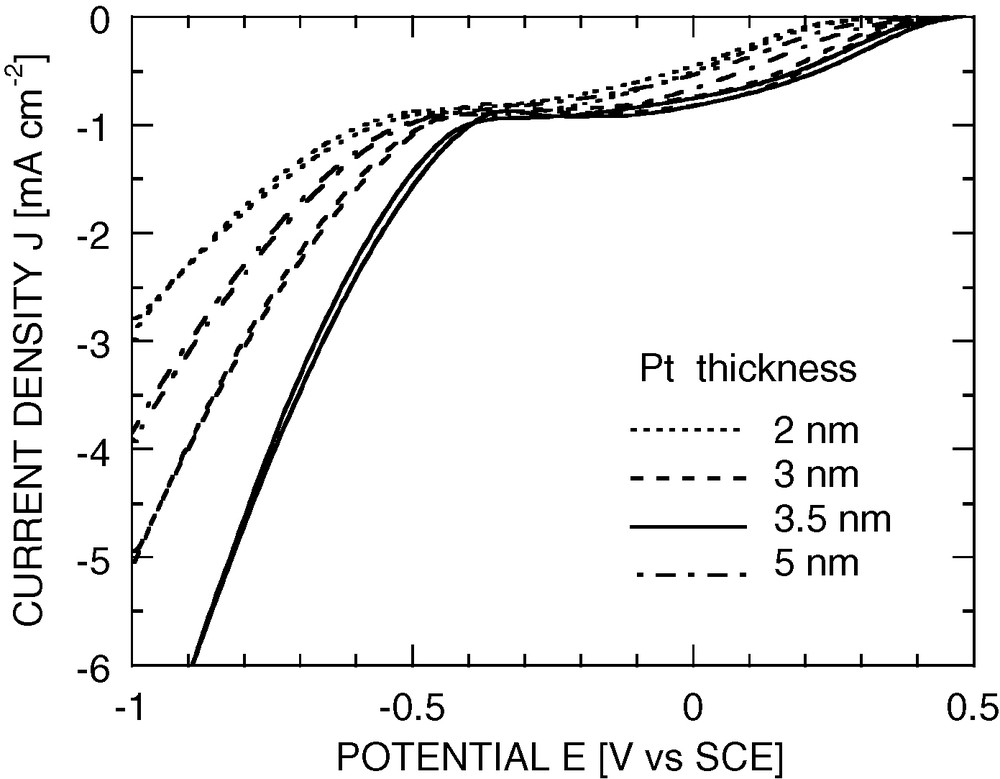
Typical cyclic voltammograms of p+/p a-Si:H electrodes coated with polyvinyl ~10 nm and with different thicknesses of sputtered Pt in 0.05 M H2SO4 solution, 17% light intensity, speed of rotation 2000 rpm and scan rate 50 mV s−1 (the solution is saturated with dry air).
The weakness of this improvement can be attributed to the unfavourable positions of the relevant energy levels (Pt Fermi level, conduction band of a-Si:H, redox level for dioxygen reduction) on the potential scale. More specifically, in acidic medium the valence band of crystalline silicon lies at a potential on the order of + 0.5 V vs. SCE [12], within 0.1 V of the onset potential for dioxygen reduction on Pt. Hence, even with ideally good kinetics, a bare p-Si photocathode could hardly exhibit a significant photopotential for dioxygen reduction. The wider gap of a-Si:H might turn the situation somewhat more favourable (unfortunately, in that case the valence band potential is unknown and hard to determine). On the other hand, in the upper range of Pt-layer thickness, the electrode behaves as a Schottky diode in series with the Pt|electrolyte interface. A photovoltage is then expected, but it is that of the p-Si/Pt diode, which is very weak, due to the very small Schottky-barrier height (resulting from the high work function, i.e. deep Fermi level, of Pt). Finally, the case of non-connected Pt islets has to be discussed separately, since it is known that discontinuous metal deposits may exhibit properties distinct from those of continuous deposits [13]. Here, each islet may bear a non-negligible charge; hence, the Fermi level is no more imposed by the properties of bulk Pt, but rather by the kinetics of electron transfer from Si to Pt and from Pt to the electrolyte. Since dioxygen reduction on Pt starts at rather positive potentials (~ +0.5 V), the Pt islets can hardly store excess electrons, so that the p-Si surface remains under weak depletion conditions, and the photopotential is still very low.
6 Functionalised a-Si:H p+/i/n+ structures
6.1 Testing a new structure
In view of these modest performances, a new approach has been initiated. Amorphous hydrogenated silicon allows for elaborating p/i/n structures, which provide a large photovoltage. One can then dissociate the electrochemical problem (relative position of the various energy levels) from the physical problem (photoeffect) by depositing a complete p/i/n a-Si:H structure and making the chemical optimisation on the outer surface. Therefore, p/i/n structures were prepared as shown in Fig. 6. Such a strategy has already been used for powering electrochemical systems for hydrogen production [14–17].
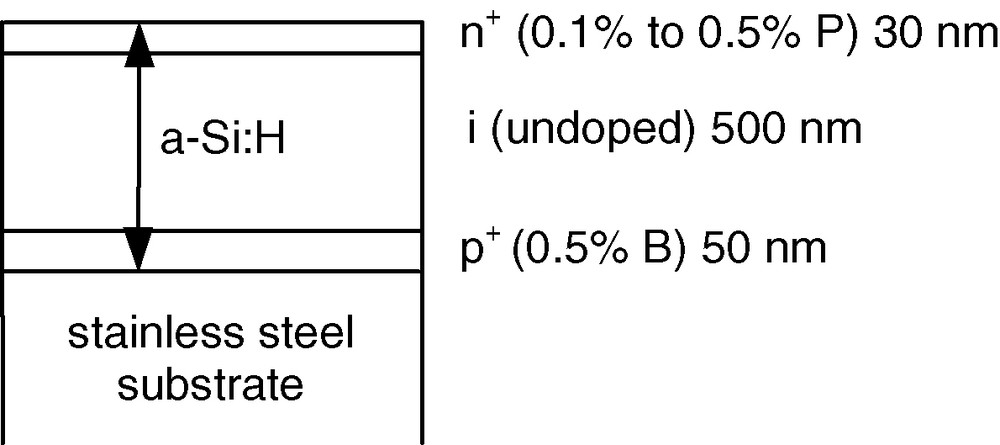
Scheme of a typical p+/i/n+ a-Si:H structure.
As expected, the voltammograms of such photocathodes were similar to those of p+/p a-Si:H structures, except for a shift toward positive potentials, corresponding to the photovoltage associated with the p/i/n structure. Highly doped p+ and n+ layers were used to maximise the photovoltage.
Similar p+/i/n+ structures were prepared and a thin platinum layer was sputtered on their surface (in that case, there is no advantage of using an electrochemical deposition of the Pt layer, because the islet morphology is not necessary: a thin continuous, semitransparent metal layer is appropriate). The Pt thickness was determined from the optical transmission spectra of a film deposited on a glass substrate in the same conditions. It appears that the film is continuous down to a thickness on the order of 5 nm, which corresponds to the optimum photocathode performance. Such a film exhibits a transmittance larger than 50% through the visible range.
In order to retain the promising performance obtained from the preceding photoelectrodes and further improve electrode stability, p+/i/n+ structures were prepared and modified using organic treatment prior to platinum deposition. Organic polymers formed anodically according to the procedures described above were found to lead to better stabilisation than simple alkyl-monolayer coating, and the thickness of the polymer was optimised. Finally, inorganic transparent conducting materials such as ITO or WO3–x, obtained by sputtering, were tested as replacements for the polymer.
Much larger improvements in photocathode performance were obtained by using such structures (a ~ + 0.9 V shift in the voltammetric curves as compared to a bare Pt cathode, i.e. dioxygen reduction starting at about +1.4 V vs. SCE in acidic medium, or at about +1.0 V in neutral medium, see Fig. 7). The coatings were again optimised. Typical parameters are (5-nm polyphenylene or 50-nm ITO) + 5 nm platinum. Polyphenylene and ITO were both found to provide good electrode stability on a scale of a few days in neutral pH medium. However, ITO is not expected to be stable in acidic medium, and a polyphenylene-coated electrode was also found to lose a few percent performance after undernight operation in acidic medium. It was found that substoichiometric tungsten trioxide WO3–x may play the same role as ITO, with typically the same thickness, and may possibly be more stable in acidic medium. However, long-term stability has still to be tested.
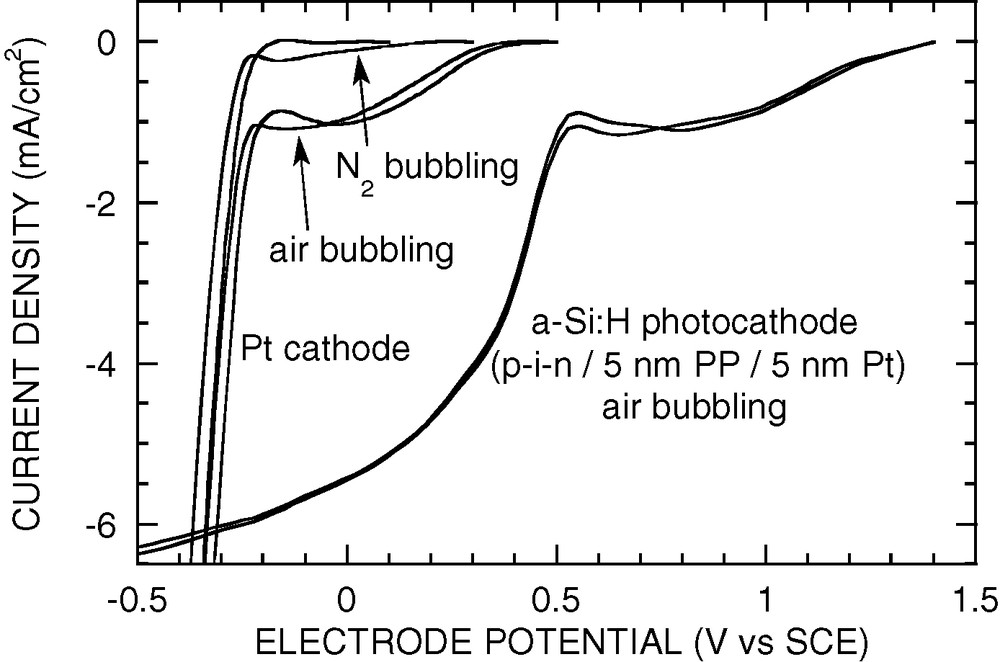
Typical characteristics of a p+/i/n+ a-Si:H photocathode coated with ~5-nm polyphenylene and 5-nm sputtered Pt in 0.05 M H2SO4. RDE 2000 rpm, air bubbling. Notice the +0.9-V shift of the voltammogram as compared to a bulk Pt cathode.
Interestingly, the organic or oxide coating has a beneficial effect, not only in terms of electrode stability, but also in terms of reliability: most electrodes prepared without such a layer exhibit no photovoltage, due to the unavoidable presence of pin-holes in the a-Si:H layer, and short-circuiting of the p+/i/n+ structure upon Pt metallisation. In the presence of the resistive polymer or oxide layer, the detrimental effect of these pin-holes is inhibited, and the fabrication yield of small-area electrodes (5 mm diameter) is nearly 100%.
6.2 Toward large-area electrodes
Large-area electrodes have been prepared according to the preceding methods for testing in an experimental purification reactor [18]. Such electrodes were made on 10-cm diameter polished stainless-steel substrates. As a further protection against short circuits, the platinum sputtering was made through a grid (80% transparency, 2-mm mesh), so that the Pt deposit consisted of non-connected millimetre-sized areas. In case a defect would be present (pin-hole) and the corresponding area would be short-circuited (despite the presence of the resistive layer), the performance of the whole photoelectrode should not be dramatically affected.
These electrodes are presently being tested in the water purification reactor. With the above precautions, the scaling of the small-area electrodes to larger size was not expected to be a big problem. The preliminary tests indicate that the expected gain in photovoltage is observed indeed. However, the geometry still has to be optimised: due to the rather high resistivity of water, it is necessary to use a thin cell, and mass-transport limitations become a problem. A solution may be to use photoelectrode patterning on a scale smaller than the thickness of the limiting layer. Also, adherence of the ITO or WO3–x films to the a-Si:H substrate appears to be a problem. We can then consider that the approach followed is feasible, but more work is still needed before satisfactory electrode stability in large-area is reached.
7 Conclusion
We have shown that it is possible to elaborate efficient and inexpensive photocathodes for the reduction of dioxygen. They are based on p+/i/n+ a-Si:H structures with a protective organic or inorganic film and a catalytic platinum coating. Though the long-term stability of such electrodes has still to be ascertained, they are readily scalable to large-area, and appear promising for the use in water purification reactors.
Acknowledgments
This work was supported by the European Community through contract No. ICA3 CT 1999-00016. Discussions with Dr. M. Etman, Dr. M. Neumann-Spallart, and Dr. G. Waldner are gratefully acknowledged.