1 Introduction
Perfluorocarbons (PFCs) and perfluoroalkylated components can profoundly alter the structure and behavior of colloids and interfaces, bubbles and membranes [1,2], these “fragile objects” that were close to Pierre-Gilles de Gennes' heart [3,4]. Perfluoroalkyl chains are also known to induce the formation of liquid crystals [5,6], another of de Gennes' favorites [7], not to speak of the specific properties that fluorinated moieties can bestow to polymers [8,9]. Our recent modest contribution to understanding the effects of highly fluorinated materials on colloids and interfaces includes investigation of surface hemimicelles [10–12], monolayer dynamics [13], emulsion film stabilization [14], bubble property control [15] and new faceted yet fluid vesicles [16]. The potential of such systems in diagnostic and therapy has also been explored, including use for cell aggregation control [17], intravascular oxygen delivery [18], pulmonary drug delivery [19] and lung surfactant replacement [20–22].
The native lung surfactant (NLS) is a lipoprotein complex that covers the alveolar surface of lungs of all mammals. By strongly reducing the surface tension at the air/water interface, NLS allows alveolar ventilation and gas exchange at physiological transpulmonary pressures and prevents alveoli from collapsing during expiration [23,25]. NLS comprises ∼85% phospholipids, ∼5% neutral lipids and ∼10% proteins (SP-A, SP-B, SP-C and SP-D) [26]. Dipalmitoylphosphatidylcholine (DPPC) is the most abundant phospholipid of NLS and is the main responsible for surface tension reduction needed for respiration. However, DPPC cannot be used alone as a lung surfactant substitute because it forms semi-crystalline domains of liquid-condensed (LC) phase at the alveolus/air interface upon compression (expiration). These LC domains do not re-spread during expansion (inspiration), thus hindering the respiration process [23–25]. In NLS, various unsaturated and anionic phospholipids and hydrophobic proteins (SP-B, SP-C) compensate for these limitations by decreasing the surface viscosity of the DPPC monolayer [27].
The absence of NLS or an alteration of its composition result in severe health conditions such as the neonatal respiratory distress syndrome (NRDS) or the acute respiratory distress syndrome (ARDS). Administration of replacement lung surfactants (RLS) from animal origin significantly reduces mortality rates in NRDS [28]. Examples of RLS include commercial preparations extracted from bovine (Survanta®, Ross Laboratories, Columbus, Ohio, USA) or porcine (Curosurf®, Chiesi Pharmaceutici, Parma, Italy) lungs [29]. ARDS is another severe lung condition that shares many of the symptoms of NRDS. However, contrary to NRDS, ARDS does not respond positively to RLS therapy because it is a more complicated pathology than that caused by the absence of surfactant only [28]. In ARDS, substantial leakage of plasma proteins, such as albumin, C-reactive protein, hemoglobin and fibrinogen, and of inflammatory mediators occurs in the alveolar spaces [30,31]. The lack of efficacy of RLS therapy in ARDS results from the fact that plasma proteins and mediators exasperate NLS function deterioration [32,33]. Numerous in vitro studies have demonstrated the surfactant inhibition properties of plasma proteins, in particular albumin [34,35]. It has been shown that serum albumin exercises a concentration-dependent surface pressure that can exceed the re-spreading pressure of collapsed monolayers in vitro [28]. As a consequence, the collapsed surfactant monolayer cannot re-spread effectively during expansion, leading to higher minimum surface pressure and to alteration of the compression/expansion isotherms. It was concluded that the re-spreading pressure may be as important as the minimum surface tension attainable in the design of new replacement surfactants that could be beneficial in ARDS therapy [28].
Because of their high biological inertness, remarkable ability to solubilize oxygen and extremely low solubility in water, PFCs have potential in medicine and biomedical research [36–42] PFCs have been investigated for intravascular oxygen transport [37] and for the stabilization of gaseous microbubbles used as contrast agents in ultrasound imaging [38]. Partial liquid ventilation (PLV) with PFCs has been explored as a treatment of the respiratory distress syndrome [43–46]. Improved oxygenation and lung compliance were achieved in preterm animal models [43], as well as in premature infants [44,45]. PFC-based PLV has also been reported to have anti-inflammatory effects in the alveolar environment of trauma patients, which may contribute to the protective role of PFCs in injuries associated with local activation of inflammatory processes [47,48]. Delivery of vaporized PFCs to oleic acid-injured ARDS sheeps has resulted in significant and sustained improvement of gas exchange and lung compliance [49,50]. As these results suggest that PFCs may be useful in pulmonary disease therapy, we have recently achieved studies aiming at determining the influence of PFCs on lung surfactant or lung surfactant models [20–22].
We report here the modifications brought by of a perfluorocarbon gas, perfluorooctylethane (C8F17C2H5 diblock, PFOE) to the compression isotherm of a DPPC Langmuir monolayer taken as a simplified model of LS film and its fluidizing effect on the physical state of this monolayer. PFOE has been chosen on the basis of preliminary observations that ranked it among the most effective PFCs in preventing DPPC crystallization [22]. PFOE has also been selected as the continuous phase of FC-in-water emulsions and microemulsions [51,52], reverse water-in-FC emulsions [53], and topical gels [54]. In each experiment, the atmosphere above the monolayer was nitrogen, or nitrogen saturated with PFOE. A second part will describe the evaluation of the effect of gaseous perfluorooctyl bromide (C8F17Br, PFOB) on a DPPC monolayer compressed over a sub-phase containing bovine serum albumin (BSA). PFOB has been chosen because it has been extensively investigated for intravascular oxygen transport [37,40] and liquid ventilation [45].
2 Experimental part
2.1 Materials
Perfluorooctylethane and perfluorooctyl bromide were kindly provided by Alliance Pharmaceutical Corp. (San Diego, CA, USA). l-α-l,2-Dipalmitoyl-sn-3-glycero-phosphatidylcholine (DPPC, purity >99%) and bovine serum albumin (BSA, essentially fatty acid free, purity >96%) came from Sigma. Spreading solutions of DPPC (1.0 mmol L−1) were prepared in analytical grade chloroform. Water was purified using a Millipore system (pH = 5.5; surface tension: 72.1 mN m−1 at 20 °C; resistivity: 18 MΩ cm). The fluorescent dye (2-[6-(7-nitrobenz-2-oxa-l,3-diazol-4-yl)amino]hexanoyl-l-hexadecanoyl-sn-glycero-3-phosphocholine, NBDC6-HPC) was purchased from Molecular Probes (Eugene, OR, USA). It was used at a lipid mole ratio of 1%.
2.2 Compression isotherms
Surface pressure (π) vs. molecular area (A) isotherms were recorded on a Langmuir minitrough (Riegler and Kirstein, Potsdam, Germany) with a working surface area of ∼120 cm2 and a sub-phase volume of ∼100 mL. The trough was equipped with two movable barriers (compression speed: 4 Å2 molecule−1 min−1). π was measured using the Wilhelmy plate method. The trough was enclosed in a gas-tight box that was flushed with nitrogen or with nitrogen saturated with PFOE or PFOB, depending on the experiment. Saturation of N2 with the PFC was achieved by bubbling through liquid PFOE or PFOB at room temperature before being flushed into the gas-tight box. The flow rate of the gas phase (nitrogen or PFC-saturated nitrogen) was set to 1.2 L min−l. Under these conditions, the evaporation rate of PFOE and PFOB was measured to be ∼6 mL h−1. Temperature was regulated at 25 ± 0.5 °C. The errors on π and A were estimated at ±1 mN m−1 and 1 Å2, respectively. In the experiments where DPPC monolayers were compressed on water, 15 μL of the DPPC spreading solution were deposited on water and the spreading solvent was allowed to evaporate for 15 min before compression. In experiments involving BSA, 15 μL of the DPPC spreading solution were spread on a cleaned sub-phase containing BSA (0.25 mg L−1) in order to avoid pre-adsorption of the protein. The isotherms were recorded 1 h 30 min after spreading of the DPPC solution. The pH of the sub-phase was ∼6.8. The isoelectric point of BSA is 5.4.
2.3 Fluorescence microscopy
Fluorescence microscopy (FM) was achieved with the Langmuir balance equipped with an Olympus fluorescence microscope (20× power objective) mounted on a xy translation stage. An Olympus 100 W high-pressure mercury lamp was used for excitation. A dichroic mirror/barrier filter assembly was used to filter and direct the excitation light (450–490 nm) onto the monolayer and to filter out the emitted fluorescence (520 nm). The latter was collected by the objective and detected via a Hamamatsu intensified camera. The microscope was linked to the gas-tight box of the trough through an extensible gusset, allowing control of the partial pressure of the PFC. The surface pressure was kept constant during the FM experiments. The fluorescent probe NBDC6-HPC is soluble into liquid-expanded (disordered) phases, which appear bright under the microscope, and is excluded from the liquid-condensed (semi-crystalline) phases, which then appear as dark domains in the images [55].
3 Results and discussion
3.1 Effect of perfluorooctylethane on the compression isotherm and physical state of a DPPC monolayer
When compressed under nitrogen, DPPC undergoes a first order phase transition from a liquid-expanded (LE) phase to a liquid-condensed (LC) phase at a surface pressure π of ∼13 mN m−1 (Fig. 1a). The LE/LC coexistence region is evidenced by the presence of a plateau on the isotherm and the formation of discrete dark domains of LC phase, as visualized by FM. Subsequent FM monitoring of the expansion of the DPPC monolayer shows the progressive fragmentation of the continuous LC phase into discrete LC domains. The size of these domains progressively decreases, until they disappear at π < ∼5 mN m−1 (molecular area A ∼ 80 Å2). No significant variation is seen on the isotherms when the DPPC monolayer is re-compressed after expansion, which means that no significant amount of DPPC molecules is desorbed to the sub-phase during the compression/expansion cycle. The limiting molecular area (A∞) is ∼50 Å2. The surface pressure of collapse (πcoll) is ∼71 mN m−1, meaning that the minimum surface tension is close to 1 mN m−1.
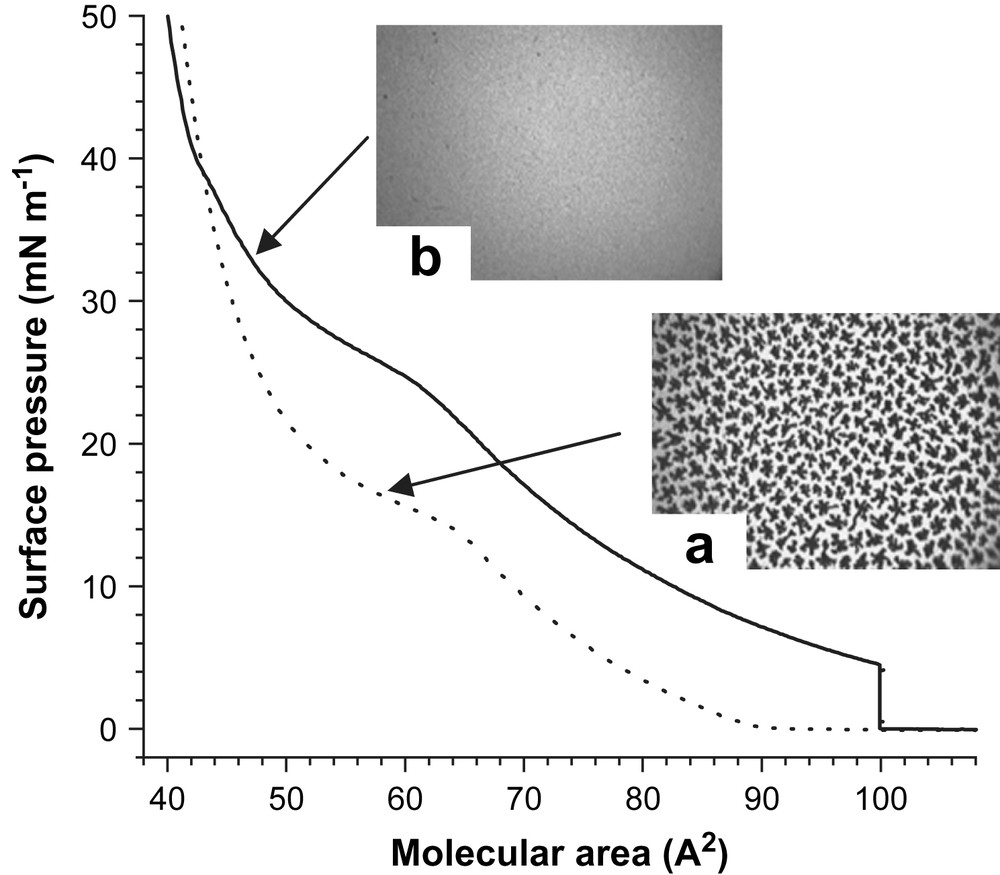
Compression isotherm of DPPC measured at 25 °C under an atmosphere of N2 (dashed line) and N2 saturated with PFOE (solid line). Fluorescence images of (a) the DPPC monolayer at 15 mN m−1, showing the crystalline domains and (b) the DPPC monolayer in contact with PFOB (30 mN m−1).
Compressing the DPPC monolayer under nitrogen saturated with PFOE changes the phase behavior of DPPC drastically. The compression isotherm is now characterized by two kinks at ∼28 and ∼38 mN m−1. Below ∼38 mN m−1, the isotherm is shifted towards the large molecular areas, indicating that PFOE molecules are incorporated into the DPPC monolayer. The transition seen at ∼28 mN m−1 is no longer of the LE/LC type, as assessed by the FM images that are bright and featureless up to 38 mN m−1 (Fig. 1b). This suggests a conformational change of the PFC molecules inserted in the DPPC monolayer. For π > ∼38 mN m−1, the isotherm becomes steeper and the limiting area (∼50 Å2) is similar to the limiting area of DPPC when compressed in the absence of PFOE. This indicates that the PFOE molecules are progressively squeezed out from the DPPC monolayer. At high π values, the FM images show the presence of very small crystalline domains, suggesting that the LE/LC transition occurred at ∼38 mN m−1. Upon expansion, below 40 mN m−1, the isotherm is shifted towards larger molecular areas, establishing that the PFOE molecules are reincorporated into the DPPC monolayer, thus increasing the re-spreading properties of the DPPC molecules, as assessed by FM. The DPPC monolayer contacted with PFOE was stable until ∼71 mN m−1 and no significant loss of DPPC molecules to the sub-phase occurred during the compression/expansion cycle. These experiments demonstrate that the PFOE molecules interact dynamically with DPPC molecules and prevent the formation of the LC phase, and hence, induce a fluidizing effect in the monolayer. It is likely that the PFOE molecules ejected from the DPPC monolayer re-spread on top of that monolayer. A similar reversible, pressure-induced vertical phase separation phenomenon has been observed in the case of monolayers made from a mixture of the long diblock C8F17C16H33 with a polymerizable fatty acid, 10,12-pentacosadiynoic acid (C13H27CCCCC8H16COOH) [56], as well as for mixtures of dipalmitoylphosphatidylethanolamine with C8F17C16H33, in which case the diblock molecules were shown to form hemimicelles on top of the phospholipid monolayer [13].
In order to assess the effect of PFOE on LC domains that are already formed, a DPPC monolayer is first compressed to 13 mN m−1, PFOE-saturated nitrogen is then allowed to flush the gas-tight box that encloses the trough, π being maintained at 13 mN m−1. The FM images show that, 3 min after the introduction of PFOE, the LC domains are significantly smaller (Fig. 2). After 5 min only, these domains totally disappear, indicating that the DPPC monolayer is totally fluid. These results are confirmed by grazing incidence X-ray diffraction (GIXD) experiments using synchrotron radiation that shows that the diffraction peaks due to the semi-crystalline DPPC domains disappear completely within 5 min after the monolayer has been contacted with PFOE.

Fluorescence images of a DPPC monolayer compressed at 13 mN m−1 under N2 (upper left). The images were obtained using the fluorescent dye NBDC6-HPC, which is preferentially soluble in the disordered regions of the monolayer [55]. As a result, liquid-expanded regions appear bright, while the semi-crystalline liquid-condensed domains appear dark. The temperature was 26 °C and the sub-phase was pure water. At time t0, the atmosphere of N2 above the monolayer was saturated with PFOE. The LC domains disappear rapidly. After 5 min, the monolayer is totally fluidized.
Taking together all the available data [20–22], the PFCs can be ranked according to the rate at which they achieve suppression of semi-crystalline domains, leading to the following efficacy scale:
C8F17C2H5 ≈ C8F18 > C8F17Br > C4F9CHCHC4F9≫ |
In the case of perfluorodecalin, complete dissolution of the domains is not achieved even after more than 1 h at room temperature, in spite of its slightly higher vapor pressure (13.5 torr at 37 °C). Perfluorooctane, which is comparable to PFOE in terms of efficacy, is too volatile for convenient use (v.p. of 52–64 torr at 37 °C as compared to 11.5 and 10.5 torr at 37 °C for PFOE and PFOB, respectively). PFOE and PFOB share together the presence of a lipophilic extremity that attenuates the overall lipophobicity of PFCs. Perfluorodecalin displays the most pronounced lipophobic character within the series, as expressed by a critical solution temperature in hexane that is about 40 °C higher than for PFOE and PFOB [57]. Also noteworthy is the fact that both PFOE and PFOB have positive spreading coefficients, respectively, meaning that they spread spontaneously when deposited on water, while the spreading coefficient of perfluorodecalin is negative [37]. Perfluorodecalin may also be hampered by its bicyclic, somewhat globular shape that does not facilitate its insertion into a phospholipid monolayer. One can conclude that PFOE and PFOB are essentially equivalent for practical purposes.
3.2 Perfluorooctyl bromide hinders the adsorption of a protein such as albumin
The isotherms of the DPPC monolayer spread on a BSA-containing sub-phase compressed under air are shown in Fig. 3. Upon compression, the isotherm is significantly shifted towards larger A values, as compared to the isotherm obtained on pure water. At the beginning of the compression (A ∼ 120 Å2), π is ∼20 mN m−1 in the presence of BSA, while it is close to zero on pure water. This means that BSA is incorporated into the DPPC monolayer upon compression and forms a mixed DPPC/BSA monolayer, in agreement with previous reports [58–61].

Compression isotherms of a DPPC Langmuir monolayer spread on a sub-phase containing BSA compressed under air. The first compression–expansion cycle is represented by a solid line and the second compression by a dashed line. FM images of the DPPC monolayer upon compression at (a) 40 mN m−1 and, upon expansion, at (b) 80 Å2 (25 °C).
The physical state of the mixed DPPC/BSA monolayer has been investigated by FM. For π < 30 mN m−1, FM images show a uniform, dark grey phase, very different from the bright and featureless images typical of fluid LE phases. This indicates that the fluorescent dye is not soluble in the mixed DPPC/BSA monolayer. This phase does not show any evidence of LC domains, which supports the fact that BSA has penetrated into the monolayer and interacted with the DPPC molecules, preventing the formation of LC domains. For π > 30 mN m−1, the shape of the isotherm became more similar to that of DPPC spread on pure water. The presence of numerous contiguous small LC domains that practically form a continuous LC phase is observed, which shows that BSA has been squeezed out from the DPPC monolayer (Fig. 3a). The value of A∞ determined on the second compression is similar to that measured after the first compression, which suggests that the stability of the DPPC monolayer is not affected by the presence of the BSA in the sub-phase.
Upon expansion of the DPPC monolayer, BSA is re-adsorbed at the interface, maintaining a surface pressure of 20–10 mN m−1 for A ranging from 50 to 120 Å2 (Fig. 3). Monitoring monolayer expansion clearly shows the role of BSA in hindering the re-spreading of the DPPC: at the end of the expansion (A ∼ 120 Å2), π is still ∼7.5 mN m−1, instead of ∼0 mN m−1 in the absence of BSA. In parallel, FM shows that, even at large A values (∼80 Å2), the phase is clearly not fluid (Fig. 3b). Large regions of LC phase, hardly fragmented, are then visible. In other regions, numerous small LC domains are present. By contrast, a DPPC monolayer re-expanded on pure water under the same conditions is totally fluid. This establishes that BSA re-adsorption during expansion of the DPPC monolayer prevents the re-spreading of the DPPC molecules, which, in the case of lung surfactant, hinders lung function [28].
The isotherms of a DPPC monolayer spread on a BSA-containing sub-phase and compressed under a PFOB-saturated air atmosphere are presented in Fig. 4.
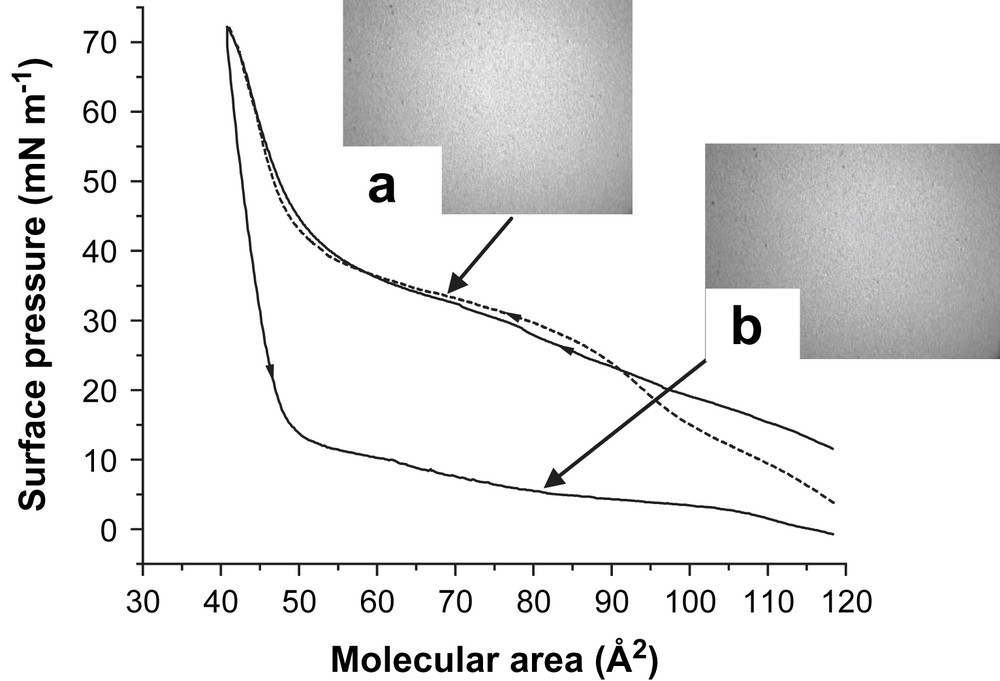
Compression isotherms of a DPPC Langmuir monolayer spread on a sub-phase containing BSA and compressed under PFOB-saturated air (25 °C). The first compression–expansion cycle is represented by a solid line and the second compression by a dashed line. Fluorescence micrographs of the DPPC monolayer (a) upon compression at 35 mN m−1 and (b) upon expansion at 80 Å2.
During the first compression, the isotherm is significantly less shifted towards large A values than the isotherm of DPPC compressed on a BSA-containing sub-phase under air. This indicates that incorporation of BSA in the DPPC monolayer is less pronounced in the presence of PFOB. It is also noteworthy that the initial surface pressure recorded with BSA in the sub-phase when the DPPC monolayer is in contact with PFOB is lower than the initial surface pressure recorded in the absence of PFOB (i.e. 12 vs. 20 mN m−1, respectively). This also demonstrates that PFOB counteracts the adsorption of BSA in the DPPC monolayer. The fact that, for π < 30 mN m−1, the FM images show the dark grey uniform phase mentioned above, indicates that the presence of PFOB limits but does not totally inhibit the adsorption of BSA. For 30 mN m−1 < π < 40 mN m−1, a bright and featureless image, typical of an LE phase, was seen (Fig. 4a). Within this pressure range, BSA has been squeezed out, while PFOB molecules are still present in the DPPC monolayer, resulting in a definite fluidizing effect. For π > 40 mN m−1, very small LC domains are visible on the FM images, meaning that the PFOB molecules are squeezed out and are on top of the DPPC monolayer. The striking point is that, contrarily to what is observed in the absence of PFOB, π decreases strongly upon expansion, even reaching ∼0 mN m−1 for the largest A values (∼120 Å2), as compared to 7.5 mN m−1 when BSA is present and PFOB absent. This means that the presence of the PFOB molecules hinders the re-adsorption of the protein upon expansion. This view is confirmed by the FM images obtained at A ∼ 80 Å2, which are uniformly bright, meaning that the monolayer is in the LE state (Fig. 4b), as compared to the FM images obtained in the presence of BSA, but without PFOB, in which LC domains are clearly present. The values of πcoll and A∞ obtained after the first compression, i.e. ∼71 mN m−1 and ∼50 Å2, respectively, show that the DPPC monolayer is not destabilized when BSA and PFOB are present simultaneously. The second compression isotherm does not show any significant difference with the first compression isotherm.
4 Conclusions
We have shown that PFOE exercises a highly effective fluidizing effect on DPPC monolayers. This PFC was more effective than perfluorodecalin and bis(perfluorobutyl)ethene in terms of fluidization of a semi-crystalline (frozen) DPPC monolayer. Data comparison indicates an advantage for PFCs that have a slight lipophilic character and a positive spreading coefficient. These features, along with a vapor pressure around 10 torr at 37 °C, appear to constitute practical criteria for the selection of PFCs destined to serve in lung surfactant replacement compositions.
The presence of small amounts of a PFC gas in the atmosphere above a Langmuir monolayer of DPPC also profoundly impedes the effect of serum albumin on the compression/expansion behavior and morphology of this monolayer. Most importantly, the isotherms and fluorescence micrographs provide evidence that the PFC gas facilitates the re-spreading of a collapsed DPPC Langmuir monolayer during expansion on a sub-phase containing bovine serum albumin. The substantial residual surface pressure (π = 7.5 mN m−1), still observed at the end of expansion (A = 120 Å2) of a DPPC monolayer when BSA is present in the sub-phase, is essentially reduced to zero when the PFC gas is introduced in the atmosphere above the monolayer. In parallel, the LC domains seen on expansion of the DPPC monolayer, even at large A values, when BSA is present, are no longer seen when the PFC is present. The observed fluidizing effect induced by the PFC thus counteracts the deleterious effect of albumin, which adsorbs at the interface during expansion, and develops a surface pressure that maintains the DPPC monolayer in a liquid-condensed state. These results suggest that combinations of a PFC gas and DPPC could be useful in the design of new replacement lung surfactants effective against the acute respiratory syndrome, in which the inactivation of the lung surfactant is largely driven by serum proteins.
Acknowledgements
The authors thank Prof. Jean G. Riess for advice and discussion. They also acknowledge the University of Strasbourg and CNRS for support.