1 Introduction
Nitric oxide (NO) produced by combustion engines, chemical industrial plants and stationary power stations contribute to different atmospheric pollution phenomena such as photochemical smog, acid rains, stratospheric ozone depletion and human health. In recent years, great research efforts have been applied to limit the emission of NO. It is well known that the selective catalytic reduction (SCR) of NO by ammonia is the world-wide used method of removing NO from stationary sources of power plants due to its efficiency and selectivity. A variety of catalysts have been developed and applied in industrial plants in order to reduce nitric oxide by ammonia to nitrogen. Excellent summaries about these studies can be found in Refs. [1,2]. In the SCR reaction, it is certain that both acidity and red-ox properties of catalysts are the controlling factors of the reactivity [3].
Catalytic tests performed with various oxide catalysts for SCR-NO with NH3 indicated that TiO2 is one of the successful supports for vanadium oxide [4]. Among the large number of catalysts active in SCR-NO, the V2O5/TiO2 is one of the most effective ones to achieve high activity and selectivity in an appropriate temperature window. In addition, a promoter such as WO3 has been generally employed in relatively large amounts (9–10 wt.%) to enhance surface acidity and the NO removal activity [5]. The industrial catalysts are constituted by V2O5–WO3/TiO2 or V2O5–MoO3/TiO2 monoliths; TiO2 in the anatase form supports a “monolayer” of V2O5 and WO3 (or MoO3) deposed by impregnation. In general, the overall surface areas of the catalysts are 50–100 m2/g, with V2O5 contents of 0.5–3 wt.% and WO3 or MoO3 contents of 8–12 wt.% Typical reaction temperature (operated generally in SCR plants) is around 350 °C [6]. Recently, it was shown that the commercial catalyst lifetime can be prolonged by sulfation with SO2 or H2SO4, the improved activity of commercial catalyst is related to the formation of new sulfate groups on the surface [7,8].
The Pillared clays (PILCs) represent a convenient source to prepare catalysts because of their low cost, high surface area and porosity, thermal stability and acidity. The number of chemical reactions for which the potential application of pillared clays, whether as catalysts or as catalyst supports, has been evaluated and is continuously increasing [9]. The use of pillared clays in catalytic reactions is reviewed, which emphasis on the possibilities offered from these catalysts to develop new processes for environmental protection, selective oxidation and refinery/biorefinery [10]. It is well known that TiO2 has a lower surface area and sinters more easily than Titanium pillared clay (Ti-PILC). It is also known that pure TiO2 anatase does not carry Bronsted acidity, while Titanium pillared clays do. The weak Bronsted acid sites arise from structural hydroxyl groups of the clay layers. Nowadays, there is no doubt that the Bronsted acid sites are the active centers for the SCR-NO reaction. Therefore considerable effort has been made to investigate Ti-PILC and their SCR behavior. Recently, many studies showed that Ti-Pillared clays used as support for vanadium were found as excellent catalysts for SCR-NO by NH3 [11–14]. It was shown that V2O5/Ti-PILC exhibited superior performance as a novel SCR-NO catalyst compared to conventional catalysts including V2O5/TiO2 and V2O5/Al2O3 [15]. It was also proved that the SCR activity on V2O5–WO3/TiO2-PILC was higher than that on V2O5/TiO2-PILC which were highly active than V2O5–WO3/TiO2 and V2O5/TiO2, respectively; especially at higher temperature [12].
More recently we have shown that the addition of sulfate to Ti-PILC increased Bronsted acidity and also significantly the SCR-NO activity which was associated with the strength of surface Bronsted acidity [16]. Our results strongly support that the high catalytic activity for SCR-NO process on sulphated Ti-pillared clay (STi-PILC) is directly related to the extent of sulfation. We have also reported that vanadia supported on sulphated Ti-pillared clay (V2O5/STi-PILC) was more active than vanadia supported non-sulphated Ti-pillared clay (V2O5/Ti-PILC) which was more active than STi-PILC [13]. In this work, the objective was to elucidate the role of WO3 on V2O5/STi-PILC. The textural, acid and red-ox properties of the catalysts have been evaluated. The WO3–V2O5/STi-PILC catalysts were tested for SCR-NO reaction and compared to V2O5/STi-PILC having the same vanadia loading.
2 Experimental
2.1 Catalysts preparation
2.1.1 Sulfated Ti-pillared montmorillonite (STi-PILC)
The initial material used for intercalation was a volclay Na+-montmorillonite from CECA. The pillaring solution was obtained by slowly adding TiCl4 into a H2SO4 solution (3 M) under vigorous stirring. Final concentration of 0.82 M in titanium and 0.2 M in H2SO4 were reached by adding water, an H+/Ti value of 0.24 was thus obtained. The fresh pillaring solution was then added drop wise to 500 mL of a suspension containing 2 g of clay to obtain sulphated Titanium pillared clay (STi-PILC), in such quantities that the final Ti/clay ratio of 10 mmol/g was obtained [16]. After 24 h of stirring, the solid fraction was separated by centrifugation, then washed several times with distilled water and dried at room temperature.
2.1.2 Vanadia supported by STi-PILC
The vanadia solution and the V2O5/STi-PILC catalyst were prepared at room temperature, as reported in Ref. [13].
2.1.3 Tungsten and vanadia supported by STi-PILC
The tungsten and vanadium solution was prepared at room temperature by dissolving ammonium metatungstate (NH4)6H2W12O40 and ammonia vanadate NH4VO3 in water acidified by oxalic acid. Tungsten and vanadium supported by STi-PILC, containing 9 wt.% of tungsten and 3 wt.% of vanadium were prepared at room temperature under vigorous stirring by incipient wetness impregnation of the sulphated Ti-PILC with the tungsten and vanadium solution. All the samples were then dried at 80 °C for 20 h and calcined at 400 °C for 3 h under flow of air. The temperature was raised at the rate of 1 °C/min up to 400 °C, maintained for 3 h.
2.2 Characterization of the catalysts
Chemical compositions were determined by analysis of the solutions obtained by attack of the solids by a mixture of concentrated fluorhydric and perchloric acid, then by nitric acid. Na content was obtained by atomic absorption, Ti content by colorimetry and S by gravimetry.
The specific surface areas, pore and micropore volumes of the samples were determined by nitrogen physisorption at −196 °C using a Micromeritics ASAP 2000 instrument. The samples were out gassed in vacuum during 5 h at 200 °C prior to nitrogen physisorption.
The X-ray diffraction patterns were recorded on a CGR theta 60 instrument using monochromatized CuKα radiation at λ = 1.54 Å. The position of the d001 line in X-ray diffraction gave the interlayer distance and consequently the pillar height.
The X-ray photoelectron spectra (XPS) were performed at room temperature with a Surface Science Instruments SSX-100 model 206 spectrometer with a monochromatised AlKα source, operating at 10 kV and 12 mA. Samples calcined at 400 °C for 3 h were compressed in a small cup under a 5 kg/cm2 pressure for 30 s and supported on a holding carousel. The binding energies (BE) of W4f, V2p, S2p and Ti2p lines were referenced to the C1s band at 284.8 eV.
Temperature-programmed desorption of ammonia (NH3-TPD) was performed in a micro-reactor system with an on-line mass spectrometer (GC–MS) to examine the surface acidity of the catalysts. In each experiment and before adsorption of ammonia a sample weight about 0.150 g calcined and at 400 °C was placed in the cell, evacuated at 400 °C for 2 h under a flow of helium and then cooled to ambient temperature. Pure ammonia gas (50 mL/min) was adsorbed at 100 °C for 20 min. Then after sweeping NH3 by He (50 mL/min) and stabilization of the base line of the detector, the temperature was progressively increased up to 400 °C at a constant rate of 10 °C/min. The desorbed NH3 amount was collected and trapped in a boric acid solution (20 g/L), then titrated by sulphuric acid (5 × 10−3 M) for quantitative determination.
Temperature programmed H2-TPR was performed to examine the redox ability of the catalyst. TPR was measured by monitoring hydrogen consumption from a hydrogen dilute in inert gas mixture (5% H2/He) while increasing the temperature of sample at a constant rate 10 °C/min and maintaining a constant flow rate (60 mL/min). The inlet and outlet gas composition were measured using a quadrupole mass spectrometer QMC 311 Balzers coupled to the system.
2.3 Catalytic activity measurement
The SCR experiments were carried out in a tubular continuous-flow fixed-bed reactor between 100 and 400 °C operating under atmospheric pressure, with a GHSV 46.000 h−1. The total flow rate was controlled by flow meter (100 mL/min). The gas mixture was: NO (0.1 vol.%), NH3 (0.1 vol.%) and O2 (2.5 vol.%) in He. The catalyst amount of 0.150 g calcined at 400 °C and the space velocity were kept constant for all experiments. Each catalyst was heated for 1 h at 100 °C under helium before adding the charge. All reported conversions were measured at steady state, under our experimental conditions, after 1 h at a given temperature. They were analyzed using a quadrupole mass spectrometer (QMC 311 Balzers).
3 Results and discussion
3.1 Characterization of the catalysts
The STi-PILC, used as a support for vanadia and tungsten, exhibited a high basal spacing of 22 Å with a large surface area of 226 m2/g and pore volume of 0.170 cm3/g significantly higher than those of the initial clay (Na+-Mont.) indicating a successful pillaring process (Table 1). The chemical compositions of the initial clay and the sulphated Ti-pillared clay support are also reported in Table 1. The increase of TiO2 and sulphur contents at the detriment of Na2O content results of pillaring and indicates the incorporation of sulphated Ti-polycations between the silicate layers upon pillaring. Table 2 clearly shows that a significant loss of surface area and micropore volume occurs upon vanadia addition. This effect can be explained by the blocking of small pores due to the building up of the vanadia layer. The basal spacing is not significantly affected by impregnation of the support with the vanadium solution [13]. When tungsten and vanadium were deposited at the same time, the surface area also decreased as sharply as the catalyst with only vanadium. The comparison of the thermal stability of the samples was performed under similar operating conditions (Table 2). For STi-PILC, the BET surface area, total pore volume and micropore volume decreased with the increase of the thermal treatment temperature but remained appreciable after calcinations at 400 °C. The decrease of the BET surface area is mainly due to the dehydratation and to the dehydroxylation of the Ti pillars and the sulfate species. Since microporosity is introduced by pillaring, the decrease of the micropore volume indicates a sintering of small pores upon calcinations. In contrast, for the samples containing vanadium and tungsten, the BET surface areas and micropore volumes increased after calcinations at 400 °C. This result could be explained by the structural changes occurring during the heat treatment. The dehydroxylation of vanadia and tungsten species with the increase of the calcinations temperature to 400 °C induces a discharge of small pores filled with vanadium and tungsten species leading to an increase of the surface areas. For all samples, the surface areas are above 150 m2/g at 400 °C; characterizing the thermal stability of the investigated catalysts.
Structural, textural and chemical analysis of the initial clay (Na+-Mont.) and sulfated Ti-pillared clay (STi-PILC) support.
Samples | d001 (Å) | SBET (m2/g) | Vp (cm3/g) | Vμp (cm3/g) | Na2O (wt.%) | TiO2 S (wt.%) | S (wt.%) |
Na+-Mont. | 12 | 40 | 0.075 | 0.002 | 3.06 | 0.33 | 0 |
STi-PILC | 22 | 226 | 0.170 | 0.046 | 0.21 | 39 | 1.1 |
Textural properties of the samples investigated.
Samples | Non-calcined | Calcined at 400 °C | ||||
SBET (m2/g) | Vp (cm3/g) | Vμp (cm3/g) | SBET (m2/g) | Vp (cm3/g) | Vμp (cm3/g) | |
STi-PILC | 226 | 0.170 | 0.046 | 154 | 0.130 | 0.025 |
V2O5/STi-PILC | 72 | 0.091 | 0.014 | 155 | 0.159 | 0.013 |
WO3–V2O5/STi-PILC | 81 | 0.087 | 0.011 | 159 | 0.163 | 0.015 |
Table 3 summarizes the XPS binding energies of W, V, S and Ti present on the surface of the samples. The W4f7/2 binding energy measured for the sample containing tungsten occurred at 36.5 eV and corresponds to tungsten in the 6+ oxidation state WO3 [17]. An additional BE at 247.5 eV was observed for W4d5/2 suggesting the existence of tungsten as W(VI) only [17]. No XPS peak at 32 eV characteristic of metallic W was observed. The binding energy at 517.1 eV typical of V2p3/2 was detected for all samples containing vanadium. The literature data show that the binding energy for V5+ in V2O5 is between 517.4 and 516.4 eV, while it is between 515.7 and 515.4 eV for V4+ in V2O4 [17]. Therefore, the observed values indicate that the vanadium is present on the surface of all catalysts investigated in the 5+ valence form, as V2O5. Binding energy at near 169 eV was measured for the S2 p [17]. According to the literature data, this binding energy is typical of sulphur in the S6+ oxidation state as in Na2SO4 or Fe2(SO4)3 [18]. This BE was observed for all samples investigated indicating that sulphur remain in 6+ oxidation state, in the form of SO42−, on the surface of the catalysts after vanadium and tungsten addition. The peaks at 161–162 eV belonging to sulphide and at 164 eV to elemental sulphur were not observed. The Ti-pillared clay material showed a Ti2p binding energy values around 459 eV. This suggests the presence of Ti4+, which agrees well with the data reported in literature [17]. This similar BE identical for all the samples indicates that the Ti4+ oxidation state remains unchanged before and after addition of tungsten and vanadium. Note that the intensities of the bands decreased in the case of V2p, S2p and Ti2p after W addition indicating an increasing coverage of V2O5, SO42− and TiO2 by WO3 (data not shown). The amounts of sulphur in the Ti-pillared clay catalysts measured by the atomic surface composition evaluated as S/(Ti + V + W) atomic ratio calculated from each atomic value obtained by XPS measurements are also shown in Table 3. It is clear that the surface atomic ratio of sulphur to the sum of the cation shows a large dependency with the metal impregnation. When only vanadia was deposited, the value of S/(Ti + V + W) atomic ration decreased. It could be explained by the decrease of exposed sulphate species on the surface of the vanadia catalyst. When tungsten and vanadia were deposited at the same time, the S/(Ti + V + W) atomic ratio decreased sharply. This result could be explained by the decrease of exposed sulfate species due to the larger coverage by V2O5 and WO3. In conclusion, the XPS results show that the catalysts contain the mixed oxide elements in the highest oxidation state, W(VI), V(V) and Ti (IV), most probably as WO3, V2O5 and TiO2 with SO42− species on the surface of the pillared material catalyst.
XPS data of the catalysts investigated.
Samples | Binding energy (eV) | S/(Ti + V + W) atomic ratio | |||
W4f7/2 | V2p3/2 | S2p | Ti2p3/2 | ||
STi-PILC | – | – | 169.5 (2.71) | 459.4 (4.65) | 0.582 |
V2O5/STi-PILC | – | 517.2 (1.41) | 169.3 (1.20) | 459.2 (3.13) | 0.264 |
WO3–V2O5/STi-PILC | 36.5 (0.84) | 516.9 (1.38) | 168.9 (0.43) | 458.8 (3.01) | 0.082 |
The NH3-TPD patterns of the clay samples are shown in Fig. 1. The shape of desorption pattern obtained for the STi-PILC support is very different from those recorded for the samples containing WO3 and/or V2O5. However, the NH3-TPD spectra of these solids contain at least two maxima. The first peak is centered at about 230 °C, while the second one, much broader, at temperature above 300 °C. This ammonia desorption suggest that there are at least two distinct NH3 species adsorbed on the sulphated Ti-pillared clay surface. The low temperature peak can be related to the desorption of ammonia either physisorbed or linked to weak Bronsted acid sites, while the second peak at high temperature can be attributed to NH3 desorbed from Lewis acid sites. It is known that the acidity of STi-PILC is essentially related to the presence of sulfate. In our previous work we have shown that the addition of sulfate to Ti-pillared clay increased significantly the total acidity and essentially the Bronsted acid sites [16,19,20]. The addition of vanadium into the STi-PILC increased the intensity of the first peak which correspond to the amount of weakly adsorbed ammonia and decreased the quantity of stronger chemisorbed NH3 molecules. Thus the addition of vanadia to STi-PILC significantly changed the surface acidic properties of the support. This is in good agreement with measurements reported in earlier study that the strength of Bronsted acidity increased with V2O5 loadings, whereas Lewis acidity, mainly observed on the Ti-PILC (sulphur free support), remained almost constant [21]. For the catalyst containing WO3 and V2O5 the major desorption of NH3 occurred between 150 and 300 °C, the peak due to weak acid sites was intense and centered at about 220 °C with a decrease of the intensity of the second peak. The change in the TPD profiles can be explained by the progressive transformation of the surface acidity after V2O5 and WO3 addition. When WO3 and V2O5 exist simultaneously on the surface of STi-PILC, the amount of Bronsted acid sites further increases, along with a decrease in Lewis acid sites. This suggests that the addition of WO3 and V2O5 produces more Bronsted acid sites while some of Lewis acid sites are covered by tungsten and vanadia. The Lewis acidity observed in all Ti-pillared clay samples was related to the acidic property of TiO2 [19,20]. The addition of V2O5 to STi-PILC induces an increase of the total amount of NH3 adsorbed on acid sites indicating that V2O5 contributes to the improvement of the total acidity (Table 4). In the case of the WO3–V2O5/STi-PILC sample, the slight decrease of the total amount of NH3 adsorbed may be explained by the coverage of the strong acid sites, arising from sulfate species, by relatively weak acid sites of WO3. This interpretation agrees the detection of few amount of sulphur on the surface catalyst as observed by the decrease of S/(Ti + V + W) XPS atomic ratio for the WO3–V2O5/STi-PILC sample.
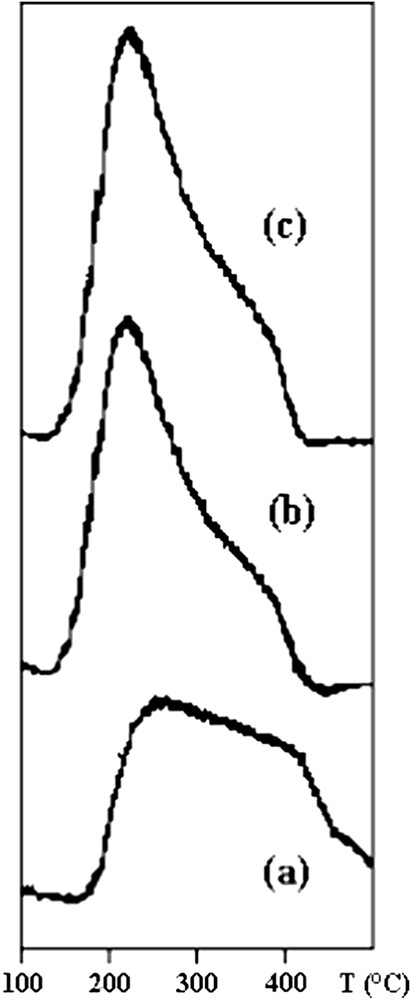
NH3-TPD profiles of: (a) STi-PILC, (b) V2O5/STi-PILC and (c) WO3–V2O5/STi-PILC.
Total amounts of ammonia adsorbed.
Samples | NH3 (μmol/g catalyst) |
STi-PILC | 175 |
V2O5/STi-PILC | 265 |
WO3–V2O5/STi-PILC | 253 |
The TPR profile of the STi-pillared clay support displays only large peak, starting near 400 °C and centered at 580 °C (Fig. 2). It was shown and well discussed in our earlier work that the hydrogen consumption over sulphated Ti-pillared clay is due to the reduction of sulfate to essentially SO2 with little H2S formation [22]. For the V2O5/STi-PILC catalyst, the threshold of hydrogen consumption began at 400 °C and two peaks were found centered at 506 and 530 °C. The first reduction peak, shifted towards lower temperature after vanadia addition, is mainly due to the sulphate reduction into essentially SO2 with few H2S and the second hydrogen consumption is attributed to the reduction of V5+ on V2O5 [22]. The reduction of V2O5/STi-PILC occurs at a temperature much lower than that of STi-PILC, showing an enhancement of sulphate reduction after vanadia addition. The TPR profiles clearly indicate that V2O5/STi-PILC is more easily reduced than STi-PILC. Since the WO3/TiO2 catalyst exhibits maxima above 700 °C, the reduction maximum at higher temperature was attributed to the reduction of TiO2 and WO3 [23]. In the case of WO3–V2O5/STi-PILC, the TPR profiles displays only a large peak, starting near 400 °C and centered at 515 °C whereas the small peak observed at 530 °C for V2O5/STi-PILC disappeared. However, the reduction of sulfate completely hides the reduction of vanadium. Therefore the modification in reduction properties of sulfate and of vanadia evidences the existence of a strong interaction between tungsten, vanadia and sulfate on the surface of the WO3–V2O5/STi-PILC catalyst leading to better red-ox properties of this material.
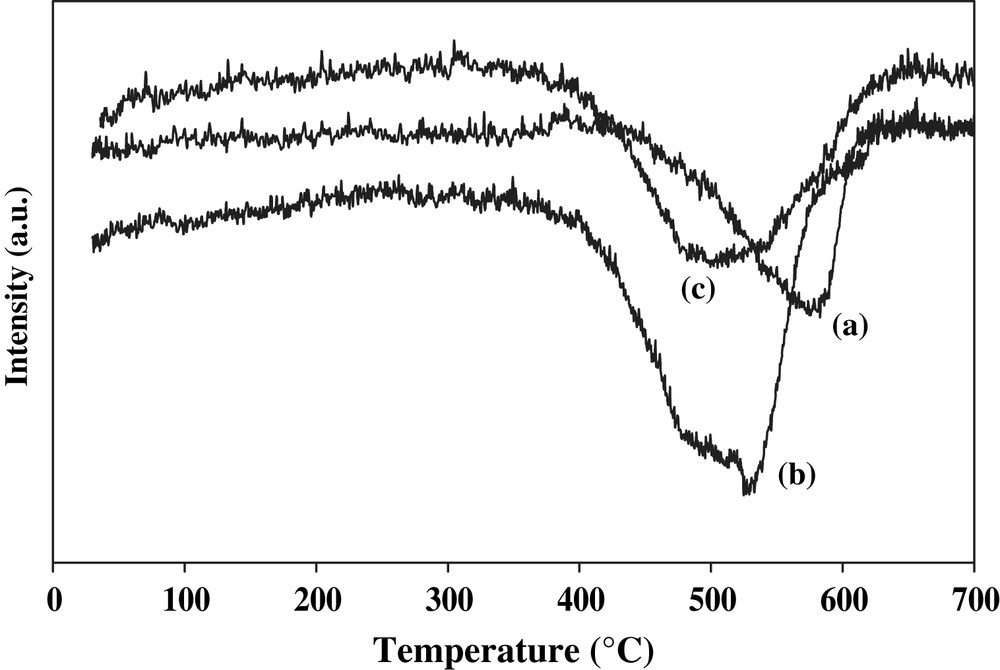
H2 consumption profile over catalysts with temperature increase during H2-TPR: (a) STi-PILC, (b) V2O5/STi-PILC and (c) WO3–V2O5/STi-PILC.
3.2 SCR-NO reaction
The NO conversions for the samples investigated are compared in Fig. 3. For the sulphated Ti-pillared clay, the NO conversion began at 200 °C and increased with reaction temperature. The SCR-NO activity of the STi-PILC support is essentially related to the presence of sulfate which enhances the Bronsted acidity of the catalyst and contributes to the improved activity at high temperature. As mentioned in our previous work, the sulphated Ti-pillared clay exhibits higher NO removal activity at high temperature than the non-sulfated ones [16]. We have shown a direct correlation between the SCR-NO activity and Bronsted acidity enhanced by the sulfation. After vanadia addition onto the STi-PILC, the SCR activity began at 100 °C, increased with temperature up to 300 °C then declined slightly at high temperature due to NH3 oxidation by O2 giving NO. Several researches have explained that the decrease of NO conversion is not caused by the decline of the catalytic activity but by the generation of NO by NH3 oxidation [1,2]. Note that no NO2 formation was observed for all the SCR experiments using our catalysts. The N2O formation was observed only on catalysts with high vanadia loading (5 wt.%) and above 300 °C as indicated in our earlier work [22].
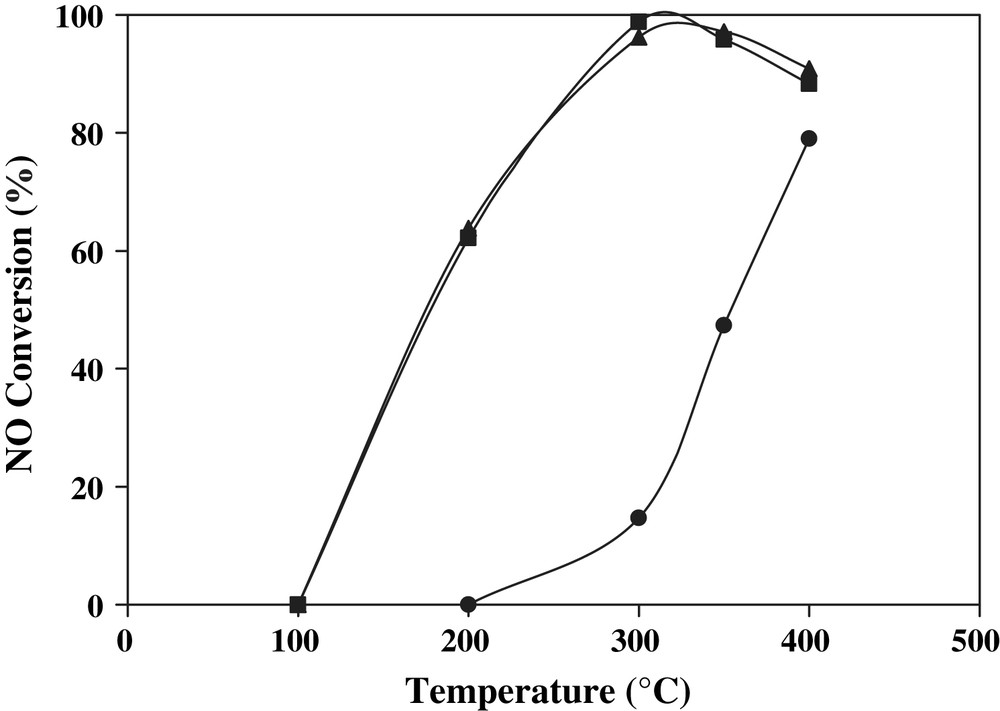
Catalytic activity for the SCR-NO reaction on catalysts investigated: (•) STi-PILC, (▴) V2O5/STi-PILC and (■) WO3–V2O5/STi-PILC.
In the case of WO3–V2O5/STi-PILC and V2O5/STi-PILC with the same vanadium content (3 wt.%), the SCR-NO activity is similar for the two catalysts, therefore the promoting effect of WO3 is not found at high temperature (Fig. 3). The identical results were observed for the same catalysts containing 1.5 wt.% of vanadium (data not shown). The highest NO conversion (97%) at 350 °C, was obtained only for the samples with 3 wt.% of vanadium. When tungsten was deposited with vanadium at the same time on the surface of sulfated Ti-pillared clay, the sulfate species seem to play a more decisive role for NO removal activity than WO3 species. These results are in contradiction with previous report results on effects of WO3 supported by sulphur free V2O5/TiO2 catalysts [2,12]. The literature also reported that the SCR-NO activities on 4.4%V2O5 + 8.2% WO3/TiO2-PILC (sulphur-free TiO2-PILC) was higher than that on 4.4%V2O5/TiO2-PILC and these two catalysts were highly active in SCR-NO than 4.4%V2O5 + 8.2% WO3/TiO2 and 4.4%V2O5/TiO2, especially at higher temperature [12]. In this later study the authors concluded that the addition of WO3 and V2O5 further increased the activities of the TiO2-PILC catalysts. From our study, it can be concluded that the contribution of WO3 on V2O5/STi-PILC to the SCR activity is negligible in presence of sulfate. Hence, when both tungsten and sulfate exist simultaneously on the surface of V2O5/STi-PILC, the sulfate species seem to play a more important role for NO removal activity than WO3.
It has been already reported that the addition of V2O5 and WO3 provides a wider temperature window than the V2O5 addition due to the improved reducibility at low temperature and the increased acidity at high temperature [5]. In this work, the surface area of all samples is similar at 400 °C. Accordingly, the change of SCR reactivity should be explained by just considering the TPD and TPR results. NH3-TPD showed that the addition of vanadium and tungsten increased the total acidy. Also H2-TPR indicated that the addition of V and W increased the red-ox properties of the material. However, the reasonable explanation of the similarity in activity of the WO3–V2O5/STi-PILC and V2O5/STi-PILC catalysts having the same vanadia loading (3 wt.%) is the dispersion between the acid and red-ox sites. TopsØe et al. studied the V2O5/TiO2 catalyst and based on TPD, TPR and FTIR they proposed two separate catalytic functions, namely acid (V5+-OH) and red-ox (V5+O) [3]. The authors suggested a dual site mechanism taken place over V5+–OH and V5+O. From this mechanism, it was suggested that the V5+O be reduced to V4+–OH by the ammonia adsorbed on the neighboring V5+–OH and V4+–OH be reoxidized by gaseous oxygen.
Now we try to compare our catalysts with those reported in the literature and especially the commercial SCR catalysts which consist of TiO2 as support and WO3–V2O5 as active phase [1,2]. Nowadays, deactivation of the commercial SCR catalysts applied in bio fuel plants is a major problem due to the higher amounts of potassium in bio fuel compared to other fuels. The commercial catalyst lifetime can be prolonged by sulfation with SO2 or H2SO4 [7,8]. Washing with H2SO4 (0.5 M) was the most effective regeneration method and the bio-modified catalyst regained 92% of its initial activity [7]. Commercial SCR catalysts containing about 0.5–1% of sulphur that mainly exists in the form of sulfate on the surface were suggested [24]. It was also shown and well discussed in our earlier work that the undesired reaction of NH3 oxidation to NO was inhibited by the sulfate species leading to an increase of the SCR-NO activity at high temperature [22].
The present study has employed pillared inter-layered clays intercalated by titanium as a support to replace the conventional TiO2, well known with a low surface area and with only Lewis acidity compared to Titanium-pillared clay (Ti-PILC). From these reports it can be suggested that Ti-PILC is a promising support to replace TiO2 for SCR catalyst and the NO removal activity can be enhanced by the increase of Bronsted acidity induced from the sulfate (1 wt.%). The experimental findings of this study suggest that the WO3–V2O5/STi-PILC or V2O5/STi-PILC catalysts may be preferably applied to SCR processes for NO containing exhaust gases from power plants which strongly require the catalysts with a high SCR activity at around 350 °C.
4 Conclusion
Vanadia enhanced the NO removal activity of STi-PILC, while no significant effect of tungsten was observed for WO3–V2O5/STi-PILC catalyst. When both tungsten and sulfate exist simultaneously on the surface of vanadia supported sulfated Titanium pillared clay, the sulfate species seems to play a more important role for NO removal activity than tungsten. As a result, the promoting effect of WO3 in the presence of sulfate (1.1%) is almost not found.