1 Introduction
Aerogels are highly porous, high surface area materials in which the solid phase is composed of a nanoscale three-dimensional network. The interconnected mesoporosity enables molecular access and provides for rapid mass transport. Aerogels have long been known for their excellent thermal insulation properties and their use as heterogeneous catalysts [1] but, until recently, their electrochemical properties had received relatively little attention. The development of sol-gel synthesis methods for transition metal oxides, pioneered by Livage and co-workers in the 1980's [2], coincided with a growing interest in using transition metal oxide xerogels as electrode materials for batteries and fuel cells as well as for electrochromic display devices. Once effective sol-gel synthesis routes were available, it then became possible for research groups to explore the question of whether transition metal oxide aerogels would be comparable to xerogels or, because of their unique morphology, possess interesting properties of their own.
The extensive research carried out by Livage and co-workers on the chemistry, structure and properties of vanadium oxide xerogels provided the basis for our work on the corresponding aerogels. The research on gel synthesis by the Livage group was particularly important for our aerogel work as the fundamental understanding of hydrolysis and condensation reactions occurring for various molecular precursors enabled us to prepare materials over a range of conditions which led to different morphologies. The electrochemical studies that were carried out on V2O5 xerogels were very important not only because they suggested that aerogels would be electrochemically active but also because the role of structural water was well identified. However, what the early xerogel work could not anticipate was how the electrochemical properties of vanadium oxide aerogels would be distinctly different from those of xerogels. As we came to realize from our studies, because aerogels are essentially all surface, the corresponding electrochemical reactions are dominated by interfaces and surfaces leading to a variety of unexpected – and important – electrochemical properties. These properties are absent in xerogels because of the low surface area (< 10 m2/g) of these materials.
This paper reviews the synthesis and electrochemical properties of vanadium oxide aerogels. We begin the review with a discussion of aerogel synthesis and structure, features which are derived directly from the early Livage studies on xerogels. In the section on electrochemical properties, we emphasize the novel properties which result from the aerogel nanostructure and demonstrate the truly exceptional energy storage properties of this material. At the conclusion of the paper, we present recent work which points to still greater levels of energy storage through the use of a low potential reactivity mechanism.
2 Synthesis and structure of vanadium oxide aerogels
The synthesis of aerogels is derived from the preparation routes developed for V2O5 xerogels. The essential difference in synthesis between aerogels and xerogels occurs after the wet gel is formed. Aerogels use solvent removal methods, typically supercritical drying, which preserve both the solid and porous networks of the wet gel so that the resulting solid phase retains the high porosity, low density and high surface area of the wet gel [2]. Thus, we can expect that the structure and morphology of the wet gel will resemble that of the aerogel. In contrast, xerogels have their pore solvent removed under ambient conditions. The capillary forces generated on drying lead to pore collapse and the development of ordered phases [3].
2.1 Synthesis routes
The two principal routes for synthesizing vanadium oxide aerogels involve the use of polyvanadic acid or vanadium alkoxides as precursors [4,5]. The first vanadium oxide aerogels were prepared from VO(OEt)3 and were based on the supercritical drying of ethanol to remove the solvent phase [6]. The high temperatures involved in this process (> 250 °C) led to crystallization of the vanadium oxide which could not be well controlled. To overcome this limitation and produce amorphous or nanocrystalline aerogel materials, we used supercritical drying of CO2 (∼42 °C and 10 MPa). In this approach, we formed the wet gel by hydrolyzing the vanadyl triisopropoxide precursor, VO(OC3H7)3, in an acetone/water mixture [7]. This synthesis method requires an intermediate step in order to replace the initial pore solvent, which is immiscible with liquid CO2, with a mutually soluble solvent such as acetone. We preferred this synthesis route because of the ability to vary the solvent : precursor ratio and control the surface area, pore volume and density of the resulting aerogel. The highly networked aerogel structures prepared by this method have surface areas of at least 300 m2/g and pore volumes of ∼1 cm3/g [7]. A variation on this approach is to produce aerogel materials by replacing the acetone with a non-polar solvent such as cyclohexane or pentane and using ambient drying conditions [8]. In this case, the non-polar solvent phase prevents pore collapse by suppressing condensation reactions. The resulting materials are somewhat denser than the supercritically dried materials with surface areas generally in the range of 150 to 200 m2/g. However, the ambient pressure synthesis is considerably easier than supercritical methods and the resulting pore size distribution seems to be beneficial for electrochemical properties [9]. This “ambigel” approach has also been demonstrated using polyvanadic acid to form the initial wet gel [10]. Another route for producing aerogels is that of freeze-drying [11]. In this case, removal of the cyclohexane solvent phase by sublimation prevents pore collapse and leads to vanadium oxide aerogels with fairly high surface areas (∼250 m2/g).
2.2 Vanadium oxide aerogel morphology
We expect that the mechanisms proposed by Livage and co-workers for the hydrolysis and condensation of vanadium oxide xerogels and the development of their ribbon-like morphology can be applied to the corresponding aerogels. We noted, for example, that as-prepared gels were deep red in color, similar to what was reported for both VO(OAmt)3 and VO(OPrn)3 precursors [5]. The growth of V2O5 sols into ribbon-like polymeric species is well documented. Such ribbons are typically 1 μm long, 10 nm wide and in the range of 1 nm thick. This ribbon morphology results from condensation reactions occurring along preferred directions leading to the formation of chains of vanadium polyhedra [3]. The precise structure of the V2O5 xerogel ribbons has been well discussed and recent work suggests that the ribbons consist of bilayers separated by a characteristic interlayer distance which serves as the location for water or ion insertion (Fig. 1) [12]. The bilayers are composed of single V2O5 layers made of VO5 square pyramids in which the distance between the V2O5 layers is 2.9 Å, in agreement with X-ray diffraction (XRD) studies. The question of whether V2O5 aerogel ribbons have the same structure as that of the xerogel is unknown at this time. X-ray absorption fine structure (XAFS) indicates that the aerogel has a square pyramid configuration for the vanadium [14] but exactly how these pyramids link together has not been determined. XRD of these aerogels indicates the presence of peaks associated with hk0 planes that correspond to the two-dimensional structure of the ribbons along the layer. However, the 00l reflections are very broad and no harmonics are evident as in the corresponding xerogels. We hypothesize that the V2O5 aerogel morphology is nanocrystalline with short range order similar to that of xerogel ribbons along the ab plane, but that the random orientation of the ribbons leads to the absence of long-range order.

The structure of a V2O5 xerogel is composed of V2O5 double layers, and the interlayer spacing serves as the intercalation region for water and ions [12,13]. Reprinted by permission from Macmillan Publishers Ltd: Nature Materials, J. Livage, “Towards smart artificial muscle,” 2:297–9, copyright 2003.
2.3 Presence of interlayer water
When the V2O5 gels are synthesized in an aqueous environment, the resulting gels are hydrated and commonly referred to as V2O5·nH2O. For xerogels dried under ambient conditions, n is about 1.8 [3]. The presence of water in the vanadium oxide xerogel structure and its influence on properties are well characterized. The water occupies the interlayer sites in the V2O5 gel structure, and the degree of hydration can be determined from the interlayer distance of the V2O5 ribbons. From the water adsorption isotherm, the first water monolayer is chemically bonded to the V2O5 structure, whereas subsequent layers are simply adsorbed [3]. The removal of water from the structure upon drying is believed to proceed by the following two-step mechanism:
(1) |
The water removal is reversible up to 250 °C; beyond this temperature, the chemically adsorbed water is removed. We expect that similar behavior occurs with vanadium oxide aerogels. After supercritical drying, aerogels have n ∼2.0 [7]. The reversibility of water removal and the limiting temperature should be the same as shown in Eq. (1). However, there is little information concerning the sites occupied by water as the interlayer gap for aerogels is not well ordered.
2.4 Intercalation of molecular species
The presence of interlayer sites in vanadium oxide xerogels and aerogels leads to the possibility of intercalating a wide variety of molecular species. One of the more interesting directions for this work has been the intercalation and polymerization of organic monomers which lead to the formation of such electronically conducting polymers as polyaniline, poly (thiophene) and poly (pyrrole) within the vanadium oxide layers [3]. One objective for this hybrid materials approach is to improve the electronic conductivity of the vanadium oxide xerogels and aerogels. A related approach has been to intercalate organic cations to functionalize xerogels, which can influence the conductivity by altering the V+5/V+4 ratio [15]. The intercalation of ionically conducting polymers used as polymer electrolytes, such as poly (ethylene oxide), within the vanadium oxide layers has also been reported [16]. In general, vanadium oxide xerogels and aerogels possess a very rich intercalation chemistry. Although this paper focuses on the application of intercalation for electrochemical properties, it is evident that intercalation processes can be used to tailor vanadium oxides for a wide range of chemical, catalytic, electrical and optical properties.
3 Electrochemical properties of vanadium oxide aerogels
The search for intercalation materials for lithium ion batteries has been of interest since the 1980's [17,18]. Vanadium oxide systems have been investigated for nearly that long as these materials represent a promising direction in that their redox potential is greater than 3.0 V versus lithium. As pointed out in a recent review, a number of vanadium oxides possess layered or open structures and exhibit interesting electrochemical properties [19]. Because of their multiple valence states, vanadium oxides offer the prospect of having greater than one-electron redox leading to higher levels of energy storage than the present generation of positive electrodes in the lithium-ion battery. Among the various vanadium oxide systems, vanadium oxide xerogels, V2O5·nH2O, emerged as one of the more widely investigated Li+ intercalation materials because of the facile synthesis routes in combination with their layered oxide network [3,20]. Reversible electrochemical intercalation of Li+ ions into V2O5 gels (V2O5·1.6H2O) was reported by the Livage group in 1983 by the following intercalation reaction [21]:
(2) |
The level of intercalation seems to be dependent upon a number of factors including the morphology of the material, the hydration state of the gel (from n = 0.3 to 2.2), the presence of V4+ and such experimental considerations as electrolyte and discharge rate. For the most part, researchers generally agree that it is possible to intercalate to x ∼1.8 reversibly, leading to a capacity close to 250 mAh/g [20]. A significantly higher level of reversible intercalation, x ∼3.3, was reported previously confirming the ability of sustaining more than one-electron redox [22]. It has been proposed, however, that this material contains less than 0.5 moles of water giving rise to an amorphous oxide rather than a layered compound [20].
Aerogels lead to fundamentally different electrochemical processes because of their morphology. The high surface area of these materials ensures that surfaces and interfaces – and not the bulk – will dominate the electrochemical properties. Thus, the conventional intercalation reactions which are used to store charge in the lithium-ion battery are less likely to be important with aerogels because the amount of “bulk” is very limited. Moreover, as we have shown by using ions other than lithium, charge storage with the vanadium oxide aerogels does not seem dependent upon crystallographic considerations [23]. In the paragraphs below, we review our research on the fundamental charge storage properties and mechanisms in vanadium oxide aerogels. We have come to realize that the distinguishing feature between xerogels and aerogels, namely the surface area, leads to fundamentally different charge storage processes. The pseudocapacitive reactions which develop because of the aerogel morphology offer the promise of creating materials that combine battery and capacitor properties to achieve high energy density as well as high power density.
3.1 Fundamental electrochemical properties of vanadium oxide aerogels
One of the limitations in determining the fundamental properties of electrochemically active aerogels is the nature of the electrode structure. Conventional composite electrode structures consist of the electrochemically active component (e.g., the transition metal oxide), a conductive additive such as carbon black to enhance electronic conduction, and a polymer binder [24]. This traditional electrode fabrication route is inappropriate for aerogels. The aerogel particles tend to agglomerate during the processing of these electrodes, which reduces their surface area and alters their pore network [25]. In processing the metal oxide/carbon/binder composite, a solvent is added to facilitate mixing. Removal of this solvent, especially if it is polar, leads to collapse of the porous aerogel network. The morphology changes associated with electrode fabrication were not appreciated and, as a result, the electrochemical responses reported in the initial studies on vanadium oxide aerogels did not represent the electrochemical properties of a high surface area aerogel, but rather the behavior of an agglomerated material whose pore network had essentially collapsed. For these reasons, aerogels received relatively little attention because their electrochemical properties seemed to offer no advantages over xerogels.
The ‘sticky carbon’ electrode described by Long et al. circumvents the limitations of conventional electrode structures and enables one to measure the fundamental electrochemical properties of the aerogel [26]. By mixing acetylene black and wax, it is possible to prepare a conductive electrode that effectively holds the finely dispersed, high surface area aerogel particles, exposes these particles to the electrolyte and provides electrical contact. An important feature of this electrode is that the aerogel particles are not aggregated on the electrode and the electrolyte has unrestricted access to the particles. By having ample electron, ion and solvent transport to the solid V2O5 phase, we are able to determine the intrinsic electrochemical properties of the aerogel.
The voltammetric responses for the aerogel immobilized on the sticky carbon electrode are shown in Fig. 2 [27]. In the LiClO4/propylene carbonate (PC) electrolyte, a broad and capacitive response is observed, and the intercalation peaks appear to be superimposed upon the capacitive response (Fig. 2a). The background from the sticky carbon electrode is negligible. At high scan rates, the aerogel responds as a conventional capacitor (Fig. 2b). In contrast, when the same V2O5 aerogel is prepared in a conventional electrode structure, as a composite with carbon additive and binder, we observe the characteristic intercalation behavior for sol-gel derived V2O5 materials (Fig. 2c).
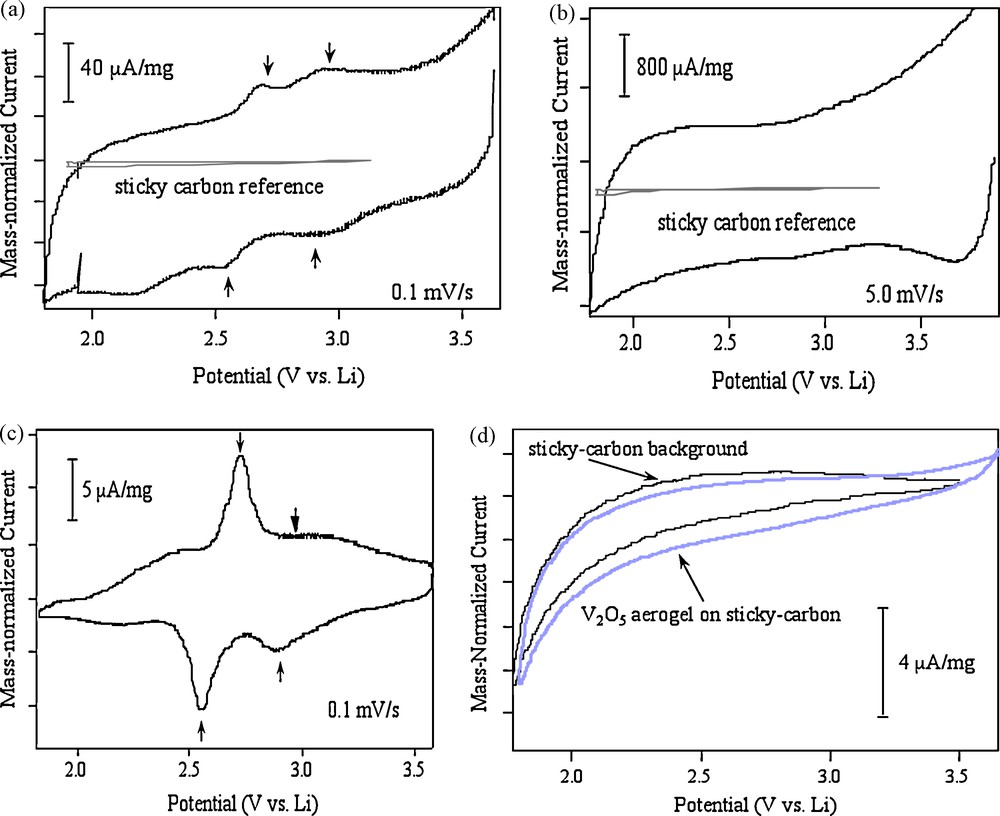
Voltammetric response of V2O5 aerogel on sticky carbon in LiClO4/PC electrolyte at (a) 0.1 mV/s and (b) 5 mV/s. The sticky carbon response is also shown. When prepared as a typical composite electrode, the response is that of (c) at 0.1 mV/s. In TBAP electrolyte, the aerogel on sticky carbon response changes as shown in (d) [27]. Reproduced with permission from Electrochem. Solid-State Lett. 3, 457 (2000). Copyright 2000, The Electrochemical Society.
The electrochemical responses for all aerogel samples can be described in terms of both a specific capacitance (F/g) and as lithium capacity (mAh/g). The former is obtained from the ratio of current to sweep rate while the latter is given by the area of the voltammetric sweep shown in Fig. 2. The data for the various samples are shown in Table 1. The lithium capacity values are in excess of 400 mAh/g, consistent with the ability to react between three and four lithium ions per V2O5. This value is nearly three times larger than the lithium capacity of a traditional positive electrode material such as LiCoO2 [17]. Such large energy density shows the benefit of working with electrochemical systems which exhibit more than one-electron redox reactions. The specific capacitance values range from 960 F/g to over 2000 F/g depending upon the drying method, supercritical or ambient. The largest specific capacitance, 2300 F/g, was obtained using cyclohexane as the drying solvent and corresponds to nearly 1500 μF/cm2. Such a large magnitude of specific capacitance arises from pseudocapacitive behavior [28], which is a very different charge storage mechanism than that which occurs with traditional intercalation materials [9].
A comparison of V2O5 aerogels and ambigels shows how specific capacitance depends on the surface area and percentage porosity.
Drying solvent | Specific capacitance | Lithium capacity (mAh/g) | Surface area (m2/g) | % porosity | Total pore volume (cm3/g) | |
(F/g) | (μF/cm2) | |||||
Supercritical CO2 | 1200 | 430 | 500 | 280 | 98 | 0.50 |
Ambigel–Heptane | 1280 | 640 | 450 | 200 | 86 | 0.60 |
Ambigel–Pentane | 960 | 520 | 400 | 185 | 89 | 0.48 |
Ambigel–Cyclohexane | 2300 | 1480 | 650 | 155 | 84 | 0.87 |
Supercritical CO2 using TBA+ | 140 | 45 | 100 | 280 | 98 | 0.50 |
Electrochemical measurements which were made using an electrolyte of tetrabutyl ammonium perchlorate (TBAP) dissolved in PC show very different behaviors (Fig. 2d). In this case, the response for the aerogel immobilized on the sticky carbon is significantly less than that obtained in the Li+ electrolyte. The area-normalized capacitance for this experiment, 45 μF/cm2, is consistent with what one obtains for surface-area-normalized double-layer capacitance at smooth electrodes; the large diameter of the TBA+ (∼9 Å) provides a situation where only surface adsorption of the ion is expected. Thus, as indicated in Table 1, the substantially greater voltammetric charge that occurs using the Li+ electrolyte (430 μF/cm2) demonstrates the importance of pseudocapacitance for the V2O5 aerogel material.
The data summarized in Table 1 indicate how the aerogel morphology influences electrochemical properties. While there is a dependence of specific capacitance on the Brunauer-Emmett-Teller (BET) surface area, a more significant factor for the V2O5 aerogels seems to be pore volume. This behavior underscores the importance of pore architecture. Supercritically dried aerogels have high surface areas but include many nano-sized pores of less than 5 nm. These small pores may not be readily accessible to the electrolyte and, in that case, their contribution to the sample capacitance will be nominal. In contrast, samples dried with a low surface tension solvent at ambient pressure tend to have larger pores, in the range of 10 to 30 nm [8]. The significant level of pore volume in combination with larger size pores greatly improves the likelihood that electrolyte penetration occurs throughout the entire particle.
The sticky carbon electrode studies are important because they enable us to characterize the intrinsic electrochemical properties of the aerogel, as neither a conductive additive nor binder is needed. In these experiments, electron, ion and solvent transport are available so that the entire particle is electrochemically accessible. Under these conditions, the V2O5 aerogel displays a combination of battery-like ion incorporation and capacitor-like response. That is, the V2O5 aerogels possess a high specific capacitance, with values comparable to those of supercapacitors, as well as a reversible capacity for lithium that is substantially greater than three electrons per V2O5. Since V2O5 xerogels do not exhibit properties of such magnitude, it is evident that the origin of this behavior is associated with the high surface area of the aerogel. As discussed later, we attribute this unique electrochemical behavior to faradaic reactions associated with pseudocapacitance.
3.2 Vanadium oxide aerogel nanocomposite architectures
Battery electrodes require the addition of an electronically conducting phase to overcome the low electronic conductivity of transition metal oxides and facilitate electrochemical reactions [17]. Typically, carbon black is added and the battery electrode is a composite consisting of micron-dimension particles. The unique nanoscale morphology of vanadium oxide aerogels raises the question of whether carbon black is the best conductive additive for this system.
One of the motivations for investigating nanocomposites composed of V2O5 aerogels and single-walled carbon nanotubes (SWNTs) is that the similar morphology and dimensional scale for these two components may lead to improvements in electrochemical performance. Sol-gel derived vanadium oxide exhibits a characteristic ribbon morphology [3], which aggregates into fibers that are approximately 10 to 20 nm wide, while the SWNTs used in this study consist of bundles of nanotubes in which the characteristic bundle diameter is on the order of 10 nm. This suggested the prospect of fabricating a nanocomposite in which the high conductivity SWNTs can provide electronic conduction in the electrode without blocking electrolyte access to the active aerogel material. In view of the capacitor-like behavior exhibited by the aerogel using sticky carbon electrodes, and prior work with high conductivity metal fibers [29], it was anticipated that the V2O5-SWNT electrodes would have good performance at high discharge rates.
Nanocomposites of vanadium oxide aerogel with SWNTs were prepared by incorporating the nanotubes as part of the sol-gel chemistry [30]. The key feature in preparing the V2O5-SWNT nanocomposite cathodes was to use a suspension of SWNTs as the source of diluting solvent (acetone) in preparing the gel. The SWNTs were received in toluene that was gradually replaced with acetone under gentle heating conditions. Although some agglomeration of SWNTs occurred upon adding acetone, the SWNTs remained suspended and did not sediment over prolonged periods of time. This suspension was combined with the requisite amounts of vanadium alkoxide precursor and water to produce a wet gel. The gelled samples were washed repeatedly in anhydrous acetone and then solvent exchanged with cyclohexane. The cyclohexane was evaporated under ambient conditions to produce the final nanocomposite. Gas adsorption measurements showed that the V2O5-SWNT nanocomposite had only slightly lower surface area and pore volume compared to the V2O5 aerogel.
A series of experiments were carried out with the objective of determining the rate capabilities for the V2O5-SWNT nanocomposite electrodes. In these experiments, the specific discharge capacity (mAh/g) was measured as a function of specific current (mA/g) for two different V2O5-SWNT nanocomposite electrodes (9 and 17 wt%) and compared to a traditional electrode, V2O5-Ketjen Black (KJB), which had an equivalent amount of conductive additive (17 wt%). The data (Fig. 3) indicate an interesting trend. At relatively low specific current (112 mA/g, which corresponds to a discharge rate of C/5), the specific capacities for the different electrodes are approximately the same. At this discharge rate, it seems that kinetic limitations are insignificant and that high capacity values for V2O5 aerogels (∼450 mAh/g) are obtained regardless of the conductive additive used in the electrode. These high specific capacity values once again show how the ability to react more than three electrons per V2O5 leads to significantly higher levels of energy storage for the aerogel as compared to other positive electrode materials. At slightly higher specific currents (560 mA/g), differences begin to appear between the two types of electrodes and it is evident that the specific capacities for the V2O5-SWNT electrodes are consistently higher than that of the corresponding V2O5-KJB electrode even though they both possess the same amount of conductive additive (17 wt%). Moreover, at rates above 1120 mA/g (2C), V2O5-SWNT electrodes containing about half as many carbon nanotubes (9 wt%) exceed the performance of the V2O5-KJB that contains 17 wt% carbon black additive. At 2800 mA/g, the highest rate tested in this study, the specific capacity for the V2O5-(17 wt%) SWNT nanocomposite electrode is approximately twice as large as the V2O5-(17 wt%) KJB traditional electrode.
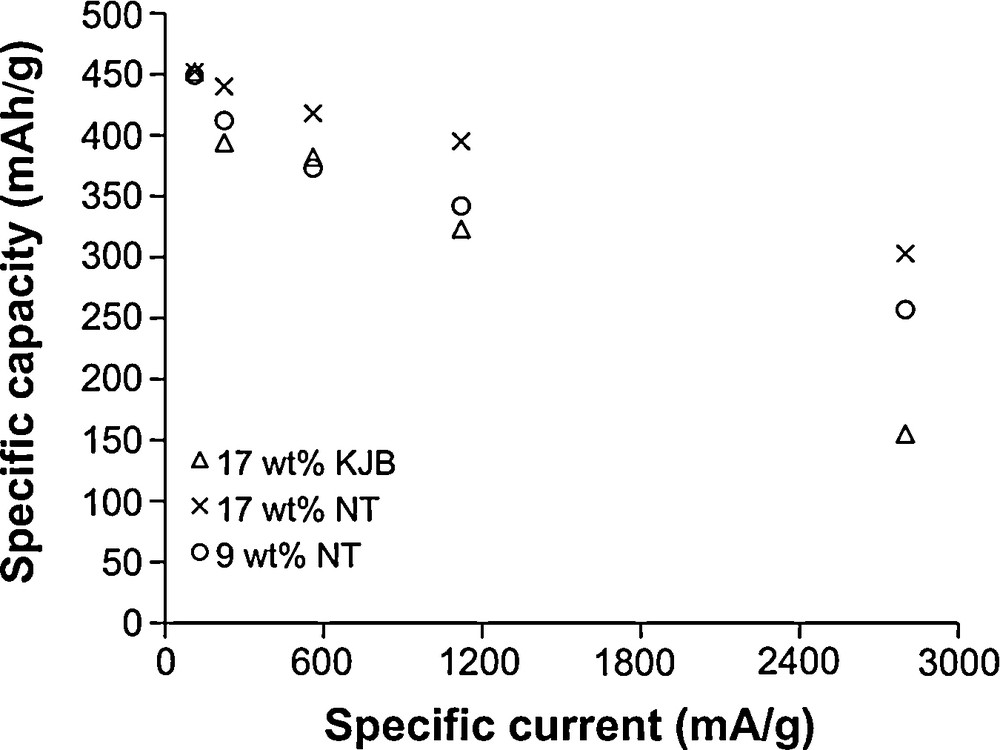
Specific capacity versus specific current for SWNT/V2O5 aerogel nanocomposites (○, ×) and conventional KJB/V2O5 aerogel electrodes (Δ). The nanocomposites show higher capacity at high specific current even when the SWNT wt% is reduced [30]. Reproduced with permission from J. Electrochem. Soc. 149, A26 (2002). Copyright 2002, The Electrochemical Society.
An interesting consideration with these discharge rate experiments is that there are no appreciable differences in the basic morphology for each type of electrode [30]. The respective pore volumes are not substantially different, which means that electrolyte access is expected to be comparable for both types of electrodes. It would seem, therefore, that the nature of the electronically conducting network and the contact developed between the conductive component and the vanadium oxide phase must be prominent factors in achieving high rate capability. SWNT may offer better electronic “wiring” to the porous aerogel morphology than carbon black aggregates. The intimate contact established at multiple locations between the V2O5 ribbons and SWNTs at the nanometer level (Fig. 4) is undoubtedly beneficial for retaining the high capacity of the vanadium oxide aerogel at high discharge rates. These results indicate that the nanostructure of the aerogel-based intercalation electrode becomes an influential factor primarily at the higher discharge rates.

Transmission electron micrograph of V2O5 aerogel/SWNT nanocomposite (dimension bar = 50 nm) demonstrates the close contact established between these two nanostructured materials [25]. Reprinted from Sci. Technol. Adv. Mater. 4, W. Dong, J.S. Sakamoto, B. Dunn, 3, Copyright 2003, with permission from IOP Publishing.
3.3 Ion insertion properties of vanadium oxide aerogels
Vanadium oxide xerogels have a rich ion exchange chemistry which enables the initial proton content of V2O5·nH2O gels to be replaced by a variety of monovalent, divalent and even trivalent ions without altering the ribbon-like structure of the xerogel [31]. Thus, it is not surprising that vanadium oxide aerogels are able to reversibly insert, by electrochemical means, a wide variety of ions which include ions larger than Li+ (Na+, K+), or of greater charge (Mg2+) or both size and charge (Ba2+). What is surprising is that the level of reversible ion insertion goes well beyond that observed with xerogels and underscores the opportunity to incorporate aerogels in alternative battery chemistries which do not involve lithium ions [23].
A summary of the electrochemical insertion properties for various ions in vanadium oxide aerogels is shown in Table 2 and includes comparisons with other forms of V2O5. The results for the largest ions, K+ and Ba2+, are perhaps the most surprising as the level of ion incorporation in the aerogel for the former is nearly four times larger than ion exchange results with the corresponding xerogels. These electrochemical experiments suggest that crystallographic changes associated with incorporating such a significant concentration of a large ion must be somehow mitigated as the electrochemical reversibility over several cycles indicates that no phase change has occurred. The results for Ba2+ seem to be the first ones to demonstrate the electrochemical reversibility of this ion in any host material.
V2O5 aerogels are able to intercalate various ions reversibly, and the capacity is higher than bulk [23].
Ions | Reversible insertion into aerogel | Comparison to bulk insertion | Comments | |
Li+ | LixV2O5 | x = 3.3 | x = 2 [32] | Much better than nanocrystalline |
Na+ | NaxV2O5 | x = 1.7 | x = 0.33 [31] | Much better than xerogel |
K+ | KxV2O5 | x = 1.2 | x = 0.33 [31] | Much better than xerogel |
Mg2+ | MgxV2O5 | x = 0.6 | x = 0.6 [33] x = 1.62 [34] | Same as nanocrystalline; less than xerogel |
Ba2+ | BaxV2O5 | x = 0.4 | N/A | Unique |
3.4 Charge storage in vanadium oxide aerogels
The electrochemical properties displayed by vanadium oxide aerogels are significantly different from those exhibited by either the corresponding xerogels or crystalline (orthorhombic) V2O5. In our “sticky carbon” electrode studies designed to determine the intrinsic electrochemical properties, the vanadium oxide aerogels exhibit both high capacity for lithium (mAh/g) as well as high specific capacitance (F/g) [27]. Such behavior, a combination of both battery-like and capacitor-like properties, is an exciting contribution to the field because it suggests the prospect of designing materials with both high energy density and high power density. In many ways, such combined battery-capacitor behavior is a ‘holy grail’ in the electrical energy storage field because battery electrode materials offer high energy density while capacitor materials provide the high power density. The goal for many years has been for battery electrodes to operate at higher discharge/charge rates and with vanadium oxide aerogels, we seem to be well on the way towards achieving this objective. Our work on the fabrication of composite electrodes consisting of SWNTs and vanadium oxide aerogels demonstrated that both high energy and power density properties are attainable in the same electrode and that we need not compromise one for the other [30]. We also determined that vanadium oxide aerogels accommodate the reversible electrochemical insertion of up to four lithium per V2O5 unit, leading to a specific capacity for lithium in excess of 500 mAh/g. This value is far greater than that reported for either vanadium oxide xerogels or crystalline V2O5, systems where lithium intercalation is well established. Such behavior, however, is not limited to lithium as vanadium oxide aerogels are able to electrochemically insert a wide variety of ions, both monovalent and divalent, at concentration levels well beyond that observed with xerogels [23].
The underlying reason of why charge storage with vanadium oxide aerogels is different from that of vanadium oxide xerogels or crystalline V2O5 is related to the fundamental morphology of the material – high surface area, interconnected mesoporosity and nanodimensional solid phase. Electrolyte penetration through the mesoscale porosity provides accessibility of molecular and ion reactants, ensuring that all of the active material can participate in the electrochemical reactions. This is clearly a significant factor in achieving enhanced capacity for lithium as well as the other ions. Associated with the porosity is the nanoscale dimensionality of the solid phase of the aerogel. These “pore walls” are very thin, typically 10 to 50 nm. Both ion and electron transport occur within these pore walls and the short path lengths for both ions and electrons are a key element in achieving the high rate discharge properties of vanadium oxide aerogels. Finally, there is the morphology of the aerogel to consider: nanoscale ribbons 10 nm wide, a few nanometers thick and microns in length. Ion intercalation into a network of empty lattice sites is the traditional explanation for charge storage in transition metal oxides and is generally well accepted for V2O5 xerogels and orthorhombic V2O5. However, there is very little “bulk” in an aerogel as the material is, for the most part, all surface. Although charge storage by diffusion-controlled intercalation behavior is likely to be involved, this contribution, by itself, is unable to explain most of the observed results. The question we need to address with the aerogels is how high surface area, well above 200 m2/g, contributes to the enhanced electrochemical properties.
As we know from the catalyst literature, high surface area provides an increased concentration of ion adsorption sites [35]. The availability of such sites is a key feature, not only for catalysis, but also for achieving energy storage through pseudocapacitive mechanisms. Pseudocapacitance is a faradaic process involving fast, reversible charge transfer processes occurring at the electrode surface [28]. This response differs from electric double-layer capacitance, a non-faradaic process in which charge is stored electrostatically from the reversible adsorption of ions onto high surface area materials. Both types of capacitance are becoming increasingly important because of renewed interest in electrochemical capacitors [36]. Recently, we showed that as the diameter of TiO2 nanoparticles decreases below 10 nm, charge storage by pseudocapacitance becomes increasingly important, leading to higher levels of charge storage than the corresponding bulk material [37]. In addition, Amatucci et al. proposed that the reversible insertion of polyvalent ions in V2O5 nano powders was partly due to pseudocapacitance [32]. These results with transition metal oxide nanoparticles provide insight concerning the charge storage mechanisms in V2O5 aerogels which possess even higher surface areas.
There are already a number of reported results indicating that V2O5 aerogels exhibit pseudocapacitive responses. In coulometric titration experiments, researchers showed that the aerogels exhibited capacitor like response (V α t) at short times which changed to a diffusion-controlled response (V α t1/2) at longer times [38]. The former is expected for a pseudocapacitance while the latter is indicative of intercalation reactions. One of the key methods for distinguishing between pseudocapacitance and double-layer capacitance is to determine the surface-area normalized specific capacitance (F/cm2). This normalized specific capacitance for pseudocapacitance is some 10 to 100 times larger than the 10 to 30 μF/cm2 expected at carbon-electrolyte interfaces [9]. In our sticky carbon experiments, we determined that the surface area normalized specific capacitance for the V2O5 aerogels was well in excess of 500 μF/cm2 for experiments carried out using lithium electrolytes. This value is a clear indication of a pseudcapacitive contribution [28]. Moreover, we were able to obtain a purely double-layer capacitance by using an electrolyte whose large cations (e.g., tetrabutyl ammonium) cannot be accommodated in the structure.
The addition of a faradaic pseudocapacitance to the traditional ion intercalation process would seem to explain the enhanced electrochemical properties observed for V2O5 aerogels. The similarity between the lithium ion intercalation process and pseudocapacitance has been discussed in detail [39]. Both mechanisms exhibit a continuous range in potential associated with their respective electron transfer processes. Thus, in standard electrochemical measurements (potentiostatic or galvanostatic), the responses for each mechanism are effectively combined. It is only when one investigates the dynamic responses that distinctions between the two contributions become apparent [37]. The increase in the level of total charge storage for lithium as well as other ions is therefore attributed to having intercalation augmented by pseudocapacitance. The fact that the latter does not have the crystallographic limitations associated with accommodating ions within the structure means that it is possible to support reversible electrochemical reactions for a range of different ions of greater size and charge than that of Li+. Finally, as we observed with TiO2 nanoparticles, pseudocapacitive charge storage leads to faster discharge/charge kinetics because charge is stored at the surface of the material rather than in the bulk. The combination of enhanced charge storage and increased kinetics observed in V2O5 aerogels enables us to consider the properties of these materials as a combination of both battery and capacitor behaviors.
3.5 New directions for V2O5 aerogels: low potential lithium reactivity
As indicated in the above discussion, the electrode materials currently being used in secondary lithium ion batteries are based on lithium intercalation reactions because of their good reversibility. One disadvantage with these intercalation materials is that because of crystallographic and structural issues, their capacity is generally limited to only one-electron redox reactions. To find new materials with greater energy density, a few research groups have begun to investigate other types of reversible electrode reactions for secondary lithium batteries. One of the most successful approaches is the use of conversion reactions for reversible electrodes [40,41].
Conversion reactions are based on electrochemically reducing a metal compound upon reaction with lithium at low potential. For a binary metal compound (MXn), with X = F, O, S, N, these reactions proceed as follows [42]:
(3) |
The high surface area, interconnected mesoporosity and nanodimensional solid phase of vanadium oxide aerogels are morphological features which may be beneficial for conversion reactions. Our experiments (Fig. 5) are the first ones to be carried out on any aerogel and therefore a key consideration in this initial work was to determine the reactivity and reversibility. The first galvanostatic charge/discharge experiments were performed at low rates of ∼C/30, based on a theoretical capacity of 1474 mAh/g for V2O5 (10 lithiums per mole of V2O5) [42]. We did not reduce the material quite that far as the capacity after the first discharge is 1340 mAh/g, corresponding to the reduction of ∼9 lithium ions. Therefore, the conversion of V2O5 to vanadium metal and Li2O did not occur. Although the exact products of this reaction have not yet been determined, one plausible explanation is that the aerogel decomposition resulted in the formation of V2O2 and Li2O. The possibility of such a heterogeneous reaction mechanism has been noted before in Ta2O5 films [44]. As shown in Fig. 5, the high first cycle discharge capacity is not entirely reversible, as there is some decrease in capacity over the first few cycles. After the first five cycles, we reach stable performance and achieve a very good reversible capacity of about 800 mAh/g (5.4 lithiums) after 20 cycles. This response contrasts significantly with the behavior of crystalline V2O5, which shows essentially zero capacity after 40 cycles at a C/5 rate [45]. While these initial results are promising because of the high energy density, it is not yet clear whether the aerogel morphology is beneficial for conversion reaction kinetics. Our experiments on the low potential reactivity of aerogels are continuing.

Capacity of V2O5 aerogel electrode cycled between 0.1 and 4 V (vs Li/Li+) at ∼C/30 rate. The high capacity results from operating at low potentials.
4 Conclusions
This review has shown that the vanadium oxide xerogel studies carried out by Livage and co-workers played a key role in the development of the corresponding aerogels. The synthesis routes, chemistry, local structure and electrochemical properties for these two materials have much in common. Indeed, the essential difference in the synthesis approaches is only the means by which solvent is removed; in the case of aerogels, great care is taken to preserve the porous morphology. V2O5 xerogels served as the initiation point for our aerogel studies as an important research objective was to address the question of how electrochemical properties change when extremely high surface areas are involved. What we found was that electrochemical responses become strongly influenced by surfaces and interfaces because of the aerogel morphology. The combination of high surface area, nanoscale solid phase, short diffusion paths and interconnected mesoporosity results in having energy storage occur through a pseudocapacitive mechanism. The significance here is that not only are higher levels of energy storage obtained, but the morphology enables high discharge rates to be realized. In this way, V2O5 aerogels serve as the basis for a new generation of electrode materials which possess the energy density properties of batteries and the power density properties of capacitors.
5 Conflict of interests
The authors have no conflict of interests.
Acknowledgements
This research was supported by the Office of Naval Research. V. Augustyn is also supported by the NSF IGERT: Materials Creation Training Program (MCTP) – DGE-0654431 and the California NanoSystems Institute.