1 Introduction
The use of membranes to selectively remove CO2 from gas mixtures is of great interest for a wide variety of applications such as upgrading of natural gas, landfill gas recovery, enhanced oil recovery and global warming prevention [1]. However, the major problem for the use of membrane-based CO2 separation is the lack of membranes with both high permeability and high selectivity. Gas separation membranes based on polymer blends were shown to have improved mechanical properties, better membrane-forming ability and higher gas permeability [2]. By blending, a combination of useful properties of each polymer can be obtained without tedious works required in the design of a new product. Compared with other modification technologies or a synthesis of entirely new materials, polymer blending has several advantages like simplicity, reproducibility and commercial feasibility [3]. However, most of the research works have been focused on polymer membrane materials involving a solution–diffusion mechanism. The performance of such materials generally falls within the trade-off relationship between permeability and selectivity suggested by Robeson [4], with an “upper bound” limit for the membrane performance.
The upper bound relationship between permeability and selectivity might be overcome with facilitated transport membranes, because the latter have both a high permeability and a high selectivity via reversible reactions between reactive carriers and the target gas, carbon dioxide.
There are two main types of facilitated transport membranes, one in which the carrier can diffuse in the membrane (mobile carriers in supported liquid membranes), the other with fixed carrier (immobilized in the membrane matrix). Although the liquid membranes with appropriate carriers exhibit generally a remarkably high selectivity and also high permeability at low CO2 pressure in the feed gas, they suffer from poor stability. For the separation of CO2, fixed carrier membranes were found to have high permselectivity as well as good stability, [5–7].
Matsuyama et al. [7] and Cai et al. [8] studied poly(vinyl alcohol) (PVA) blend membranes with polyethylenimine (PEI), and with polyallylamine, respectively, in the separation of CO2 from other gases. Nevertheless, those papers did not report on the blend membrane structures, except the crystallinity for Cai's paper. Polyallylamine contains primary, and PEI primary and secondary amine groups, that can all react with CO2 reversibly. The results showed that the selectivity of CO2 over N2 exhibits a maximum with the increase of the fixed carrier content in the blend membranes. Such a behavior was attributed to a change in the PVA crystallinity with the fixed carrier content [8]. A similar behavior was observed for other systems with mobile amine carriers. However, membranes with amine-based carriers suffered from a strong reduction in performance with the reduction in the relative humidity in the gas mixtures, because the presence of water in the membrane is required for the CO2–amine group interactions [8].
Ward and Neulander [9] investigated the separations of SO2/CO2 mixtures by liquid membranes and found that polyethyleneglycol (PEG) exhibited an excellent solubility (0.7 mol/l atm) for SO2 compared to that of CO2 (0.1 mol/1 atm) at 25 °C. Although the separation of CO2/SO2 was not effective, PEG was found to be an excellent solvent for polar gases.
Kawakami et al. [10], after a study on PEG loaded in porous regenerated cellulose, concluded that the acid-base reaction between the acidic CO2 and the electron-rich ether oxygen atoms of the PEG molecules is probably responsible for the enhanced solubility of CO2 in PEG. However, PEG as a pure polymer suffers from bad mechanical properties, and its high crystallinity reduced the permeability of polymer system containing long PEG sequence [11]. Considering the ethylene oxide as the chemical unit capable of providing a good balance of CO2 separation and permeation properties, Lin and Freeman [11] defined three strategies for incorporating high concentrations of PEO with low crystallization potential into polymers They consists in: (1) using low molecular weight liquid PEO or PEG; (2) designing phase separated block copolymers with EO-rich but short segments; and (3) building highly branched, crosslinked networks with high concentrations of PEO.
In this paper, we followed Lin and Freeman's idea of enhancing CO2 separation properties with a favorable solubility selectivity of membranes by preparing and studying blend membranes consisting of PEI as a fixed carrier for CO2 facilitated transport, and PEG as CO2 solubility enhancer, in a PVA matrix.
2 Experimental
2.1 Chemicals
PEG with the average molecular weight of 10,000 was purchased from Aldrich and used as received without further purification. PVA (Aldrich, 99% hydrolyzed derived from poly(vinyl acetate)) has an average molecular weight of 89,000–98,000. PEI with an average molecular weight of 2000 (or 25,000) was purchased from Aldrich as concentrated aqueous solutions. The ratios of primary amino groups/secondary amino groups/tertiary amino groups in both PEIs are approximately equal to 1/2/1.
2.2 Membranes
PVA/PEI/PEG membranes were prepared by solution casting and the solvent evaporation technique. 7 g of PVA were boiled in 93 ml of deionised water to form a 7 wt% solution at 95 °C. PEI and PEG aqueous solutions of 7 wt% were prepared from the corresponding polymers at 25 °C. PVA, PEI and PEG blend solutions were then prepared by thoroughly mixing the individual solutions in different ratios. We only used limpid solutions, i.e. solutions without phase separation. The bubble-free blend solution was cast to the desired thickness on a clean glass plate and the solvent (water) was allowed to evaporate slowly at room temperature for a period of 24 h. The resulting membrane was removed from the glass plate and dried in a vacuum oven at 50 °C for 12 h. The membranes were designated by their relative weight percent in the blend, with the numbers given in the same order as that of their abbreviated name.
2.3 Permeation experiments
The CO2 and N2 permeation properties of films were determined using the permeation apparatus previously described [12]. Before a measurement, the air present in the permeation cell was completely evacuated by applying a vacuum on both sides of the film for one night. The pressure in the permeation cell had a constant value < 5 × 10−3 mbar at the beginning of the permeation experiment. Then the upstream side of the permeation cell was exposed to a fixed pressure of the gas to be tested (0.5 bar < p1 < 3 bars, according to the case).
The increase in the pressure p2, in the calibrated downstream volume was measured using a sensitive pressure gauge (0–10 mbar, Effa AW-10-T4) linked to a data acquisition system.
The permeability coefficient, P, was calculated using the variable pressure method [13] assuming p1 >> p2: P = JstL/p1, with L the sample thickness and Jst the steady-state gas flux obtained from the slope of the steady-state part of the curve, p2 versus time. The accuracy on P values is ca. 6%.
The permeability coefficient P(cm3(STP) cm/cm2 s cm Hg) and the ideal selectivity α were determined by using the following equations:
(1) |
(2) |
where Q is the quantity of STP gas permeated in a time interval t in the steady state of gas flow, A is the effective film area for gas permeation, l is the average film thickness, and p1 and p2 are inlet and downstream pressures, respectively. and are the permeability coefficients of pure CO2 and N2, respectively.
2.4 Thermal behavior studies by differential scanning calorimetry (DSC)
The thermal behavior of samples was analyzed by using the conventional Differential Scanning Calorimeter Perkin–Elmer DSC-7. Samples with a mass of about 10 mg were used. The heating step was done from 0 °C to 260 °C at a rate of 10 °C/min followed by an isothermal heating for 10 min, a first cooling from 260 to 0 °C, and a second heating in the same range. All DSC runs were carried out under nitrogen atmosphere to minimize the oxidative degradation. Before all DSC experiments, the baseline was calibrated using empty aluminum pans, and the DSC apparatus was calibrated using melting temperature and enthalpy of a high-purity indium standard (156.68 °C and 28.45 J/g).
The degree of crystallinity (Xc) was determined with the following equation:
(3) |
where ΔHm is the PVA phase melting enthalpy per gram of PVA in the membrane sample, ΔHm0 is the extrapolated value of the enthalpy corresponding to the melting of 100% crystalline PVA (ΔHm0 = 140.9 J/g [14]).
3 Results and discussions
3.1 Structure analyses
3.1.1 On the polymer miscibility in blend membranes
Fig. 1 shows the scanning electron microscopy image of the cross section of different blend membranes. It can be seen that all the membranes have a symmetric structure without skin. The presence of nodules indicates a phase separation in the ternary membranes. The oblong form of the nodules may be caused by the deformation in shrinkage of the membrane due to evaporation of the solvent from the membrane during the membrane formation. The higher the PEG content, the larger the number of nodules; this suggests a poor miscibility of PEG with the other components.
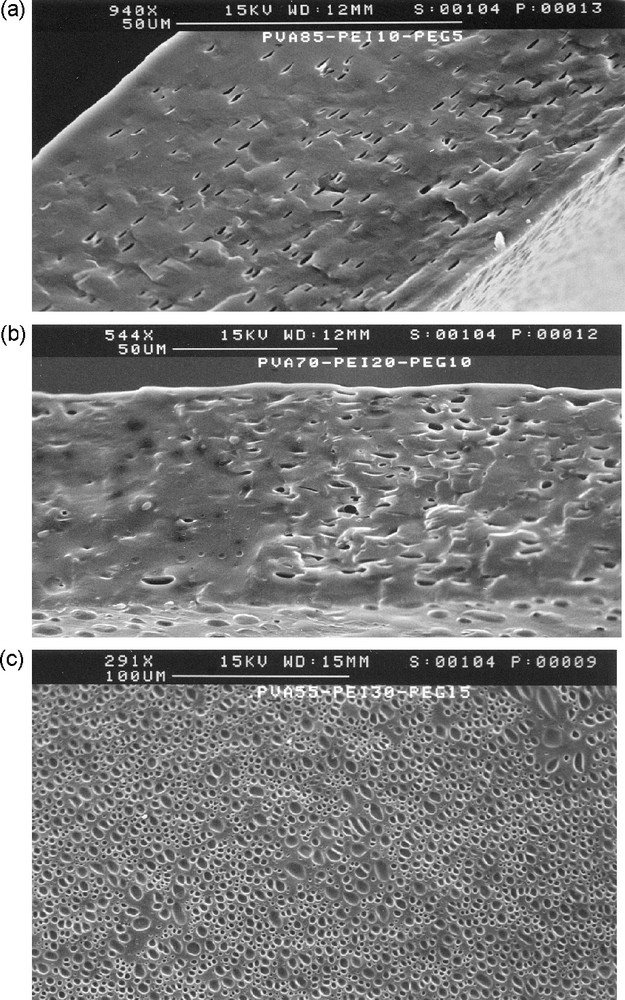
SEM images of the cross-section of three PVA/PEI/PEG membranes: (a) 85/10/5; (b) 70/20/10; (c) 55/30/15.
Phase separation may occur in the solid materials, even when the polymer components are completely miscible in solutions. In fact, the solvation molecules that screen out partially the repulsive interactions between solvated chain segments are removed upon drying, and repulsive polymer segment–segment interactions may prevail, leading to a phase separation and membrane opacity. In our case, we observed clear films of binary PVA/PEI blends in a large range of blend composition. On the contrary, PVA was not completely miscible with PEG, as opaque films were obtained at PEG contents larger than 5 wt. % in both PVA/PEG and PVA/PEI/PEG blend membranes. SEM pictures shows more and more nodules in the matrix when the PEG content increases, suggesting a separation of PEG into a dispersed phase (Fig. 1). However, membranes of good enough mechanical properties for gas permeation test were obtained up to 20 wt. % of PEG.
PVA is a semi-crystalline polymer. The crystalline state is the most stable state of a polymer; nevertheless strong interactions between the antagonist groups on two different polymers can inhibit the crystallization during the solvent removal state. We showed that strong hydrogen bonding interactions between PVA hydroxyl and Poly(vinyl pyrrolidone) (PVP) carbonyl groups can kinetically block the PVA crystallization [14,15]. The compatibility of PVA/PVP blend in the whole range of composition was proven by DSC studies, since the unique glass transition varied with composition according to the Fox-Flory's equation was observed for the blends. The blend membranes were stable enough for their use in low swelling conditions, e.g. pervaporation of organic solvents contaminated by limited water amounts [16].
Although the interactions between PVA and PEI cannot be as clearly demonstrated by infrared spectroscopy as those between PVA and PVP, where distinct absorbance peaks were observed for each polymer component, we speculate that strong interactions do exist between the PVA and the PEI nitrogen atoms. In fact, PEI nitrogen atoms which are stronger hydrogen bond acceptors (or Lewis base) than PVP, must generate stronger hydrogen-bond interactions and blend miscibility. The main IR peaks of the polymer components are given in Table 1. The better and better resolution of the broad band of strong H bonds of PVA hydroxyl in the range of 3000–3600 cm−1 into distinct peaks with the increase in PEI content in the PVA/PEI blends is consistent with the hypothesis of strong H bonds between PVA and PEI (Fig. 2a). The PVA/PEI membranes of different compositions which exhibited depressed PVA crystallinity (disappearance of the characteristic peak of PVA crystallinity at 1142 cm−1) confirm the hydrogen bond interactions between PVA and PEI that inhibited PVA crystallization and promote the polymer miscibility in the amorphous phase.
IR wavenumbers of characteristic bonds of the homopolymers (PVA,PEI and PEG).
Frequency | Bond type |
3260 cm−1 | OH stretching |
1142 cm−1 fine peak | PVA crystallites |
1100 cm−1 | CO stretching (PVA, PEG) |
3300 cm−1 broad band | Stretching of H-bonded OH (PVA) |
3300 cm−1 | NH stretching of primary and secondary amines of PEI |
1578 cm−1 and 1656 cm−1 | NH bending of PEI |
765 cm−1 and 1300 cm−1 | CN stretching of PEI |
2850/2785 cm−1 | CH2 asymmetric/symmetric stretching (PVA, PEG, PEI) |
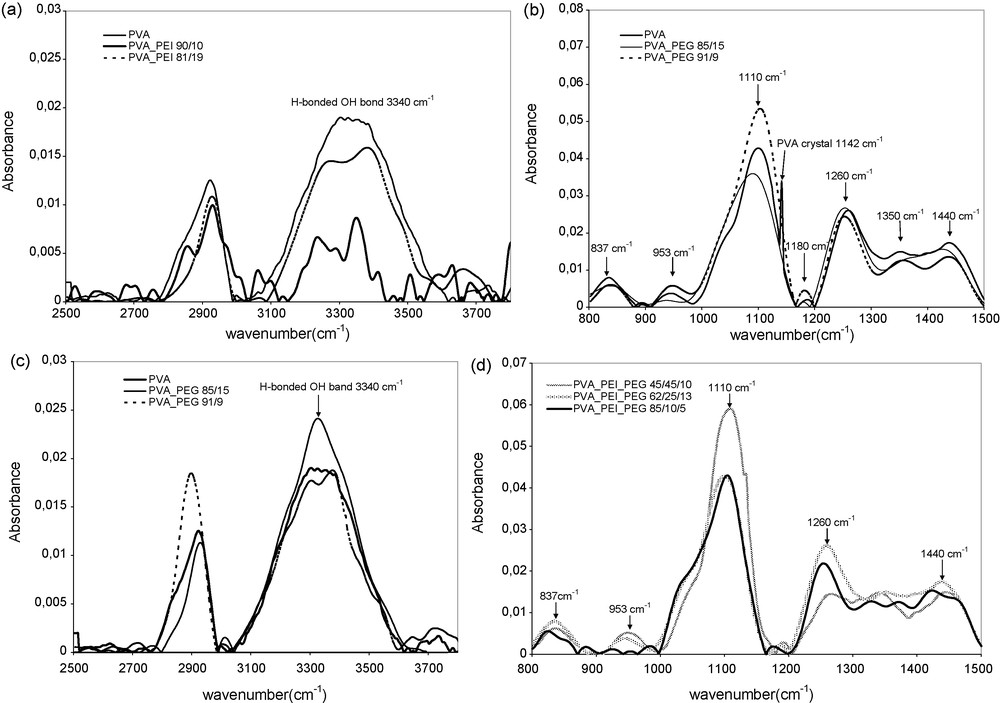
FTIR ATR spectra of PVA/PEI, PVA/PEG and PVA/PEI/PEG membranes with different composition (in wt%): (a) and (c) 2500–3600 cm−1 wavenumbers; (b) and (d) 800–1500 cm−1 wavenumbers.
PVA/PEG blends, which were only partially compatible as mentioned above, showed in their IR spectra (Fig. 2b) some proofs of polymer component interactions, e.g., the decrease in the PVA crystallinity peak at 1142 cm−1 and a shoulder that appears on the low wavenumber side of the broad OH stretching vibration band (at ca. 3260 cm−1). The broad OH stretching vibration band, which is highly sensitive to hydrogen bonds, changes with the blend composition and nature. In a previous work [14], we showed that this broad OH band in PVA/PVP blends split into three components. The component of lowest wavenumber (at ca. 3260 cm−1) was attributed to the hydroxyl groups involved in multiple hydrogen bonds in organized zones (PVA crystallites), the intermediate peak around 3300 cm−1, attributed to the OH groups bonded simultaneously to a hydrogen-bond acceptor (via H) and a hydrogen-bond donor (via O), and the component around 3400 cm−1, attributed to the mono-hydrogen-bonded OH groups. In the PVA/PEG 85/15 binary blend, the intermediate component of hydrogen-bonded OH is the strongest, while the PVA/PEG 91/9 binary blend exhibits larger multiple-bonded component and monobonded components (Fig. 2c). There were thus dominating multiple-bonded OH, e.g. those in organized zones like more or less perfect PVA crystallites and monobonded OH at lower PEG content.
The analysis of these interactions is very difficult due to the complex situation of this binary system, where the same donor and acceptor groups are present in both polymer components. We speculated that the PEG chains, which are very flexible, can accommodate the positions for which the hydrogen bonds are the most stable, i.e. those making possible a double-bonded OH. However, since there are in PEG no atoms with stronger hydrogen bonding power than those in PVA, PEG would be unable to block the formation of PVA crystallites as shown by the presence of the OH band component at 3400 cm−1. There are also some changes in the peaks around 1100 cm−1 and 2800 cm−1, assigned to CO and CC vibrations, which are difficult to analyze (Fig. 2b, 2c).
The IR spectra of the ternary blends exhibit the similar features to those of binary blends. The characteristic peak of PVA crystallinity at 1142 cm−1 disappears in the blend spectra (Fig. 2d).
PVA was chosen as the matrix of the active layer because of its good film forming, its good compatibility with different polymers due to its hydrogen bonding ability with polymers bearing Lewis-base groups, and its high hydrophilicity. The last property, which is also due to the ability of PVA to form hydrogen bonds with water, is required for a good CO2 permeability via an amine-type carrier as mentioned in the introduction. However, its main drawback is a large drop in the mechanical and gas–separation properties of the PVA-based membranes in the presence of high humidity in the feed gas [8]. To circumvent this problem, PVA can be crosslinked with various agents like aldehydes, diacids, diepoxides, di-isocyanates,… to form a semi-interpenetrating network with limited swellability and alteration of membrane properties while keeping their hydrophilicity for CO2 capture. We did not crosslink the blend membranes because of the easier reactions of the amine groups with those crosslinkers, which would greatly reduce the number of active groups available for CO2 facilitated transport [17].
3.1.2 DSC studies
The ternary-blend membranes exhibit two endothermic peaks, one at a temperature slightly lower than that of pure PVA-crystallite melting, attributed to the melting of crystalline PVA, the other at 63.5 °C. The former is attributed to the melting of crystalline PVA, and the latter attributed to the melting of crystalline PEG [18]. PEG melting peaks in the blends are sharp and stand at the same temperature as that of pure PEG, 63.5 °C, so that we can conclude that the PEG crystallization in the blends is similar as that in pure PEG (Fig. 3), i.e. not much perturbed by the presence of PVA and PEI. Such a result is consistent with the observation of pure PEG nodules in the membrane matrix.
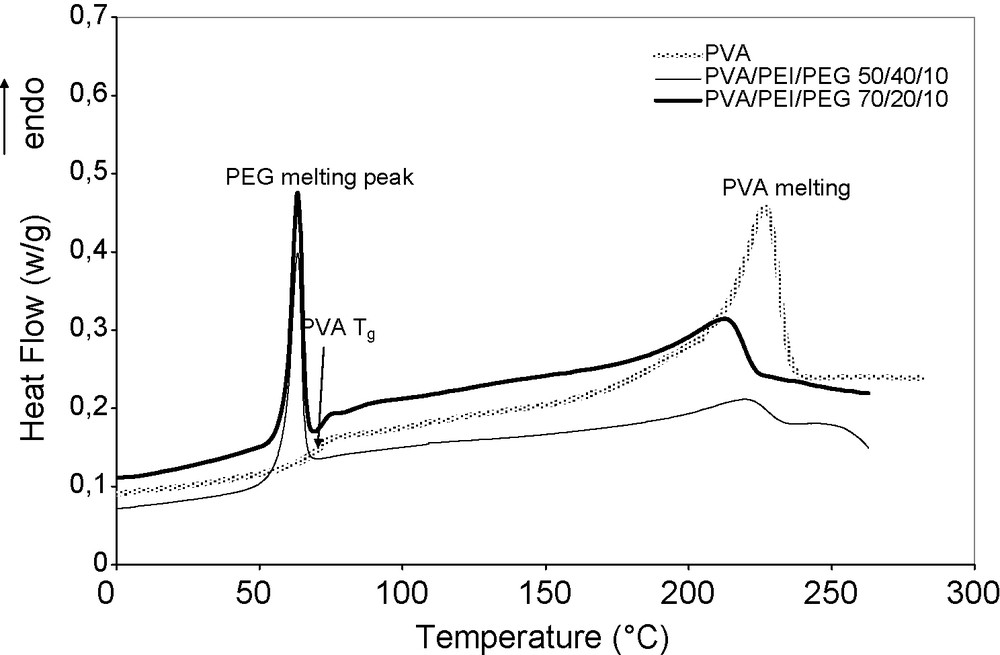
DSC thermograms of pure PVA, PVA/PEI/PEG 70/20/10 and 50/40/10 membranes, respectively.
The left shift of the PVA melting peak (towards 214 and 222 °C for PVA/PEI/PEG 70/20/10 and 50/40/10 membranes, respectively) and their broader form suggest less organized and less homogenous PVA crystallites in the blends (Fig. 4). Such a change in the PVA crystalline phase can be attributed to the hydrogen bonds between amorphous PVA and PEI (revealed in IR spectra, Fig. 2a), which perturb the PVA crystallization from amorphous PVA. We expected a significant decrease in the value of the glass transition temperature (Tg) of amorphous PVA due to its interactions with PEI, since PEI is a rubbery polymer (Tg = −25 °C). Surprisingly, the PVA Tg- value remains the same, ca. 70 °C according to Fig. 3. We do not know the origin of such a departure from the general rule.
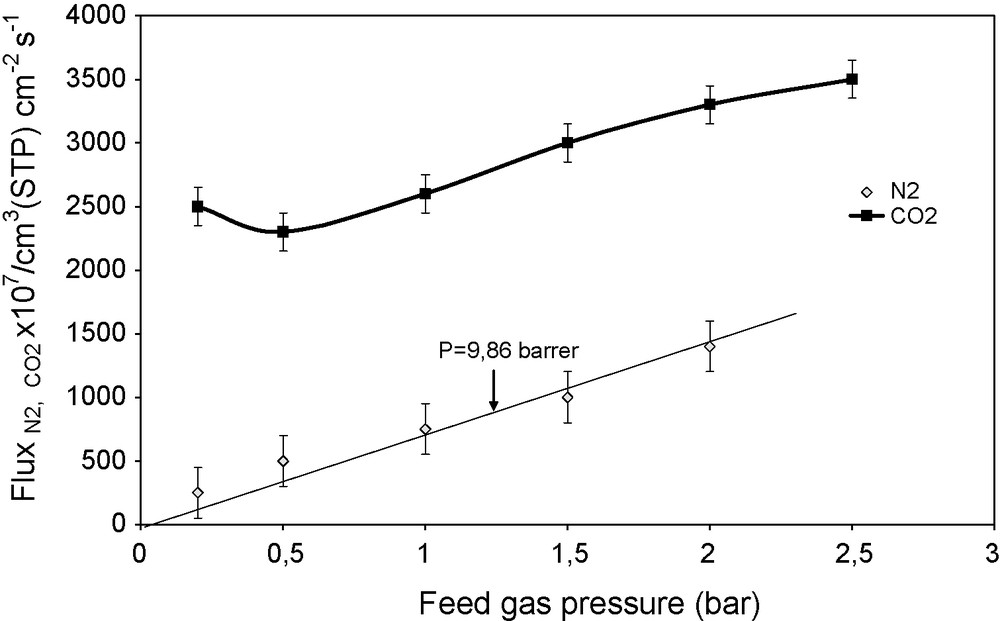
Gas flux versus feed gas pressure for PVA/PEI/PEG 60/20/20 membrane tested with pure CO2 and N2, at 25 °C.
The thermograms of ternary-blend membranes exhibit a downward baseline shift just after the PVA melting (Fig. 3), the reason for which is unclear. Such a baseline shift, in addition to the possible change in the PVA-crystallite melting enthalpy, makes the estimation of PVA crystallinity questionable. However, we believe that the PVA crystallinity in the blends was truly reduced with regard to that in pure PVA. In this respect, DSC appears to be a more suitable method for the evidence of the PVA crystalline phase than IR spectroscopy: DSC showed a certain type of chain organization in PVA crystallites, while the IR spectra indicated a disappearance of PVA-crystallites in the ternary-blend spectra.
3.2 Gas transport properties of PVA/PEI/PEG membranes
3.2.1 Effects of feed gas pressure
The permeation flux increases linearly with the feed gas pressure in the case of nitrogen, while it goes through a shallow minimum at low pressures (Fig. 4) for CO2. As the driving force for permeation increases with feed gas pressure, a decrease in the flux with the feed gas pressure increase means that the permeation does not obey the classical solution–diffusion mechanism. At very low feed gas pressures, CO2 permeability is remarkably high. One can infer that the contribution of CO2 dissolution to the total permeation in the classical mechanism is very small at low gas pressures, while the abundance of free amine groups in the membranes with respect to CO2 makes the contribution of CO2–amine reaction to the permeation high (Fig. 5). As the CO2 pressure in the feed increases, free amine groups become less and less available (progressive saturation of the carrier by CO2), making the contribution of CO2 transport via amine carrier site smaller and smaller with respect to the contribution of the normal solution–diffusion mechanism. At high feed gas pressures, CO2 permeation is dominated by the normal solution–diffusion mechanism. Gases which are not complexed by amines, like oxygen, nitrogen or methane, permeate according to the sole solution–diffusion mechanism, with a constant permeability coefficient [19] (Fig. 5). This characteristic of facilitated transport discussed in detail in ref. [20], is demonstrated by the similarity of the curves in Figs. 5 and 6 to those in Fig. 17.1 of ref. [20]. Facilitated transport membranes work on the basis of a special sorption and diffusion mechanism: the sorption into the membrane upstream, as well as the desorption from the downstream face, is due to a reversible complexation reaction, in addition to the normal gas transport by the solution–diffusion mechanism. Previous research proved that amino groups could react with CO2 according to a reaction, written here with a primary amine [21,22], whose efficiency in the CO2 capture at the membrane surface is high:
RNH2 + CO2 ⇔ RNHCOO− + H+ |
RNH2 + H+ ⇔ RNH3+ |
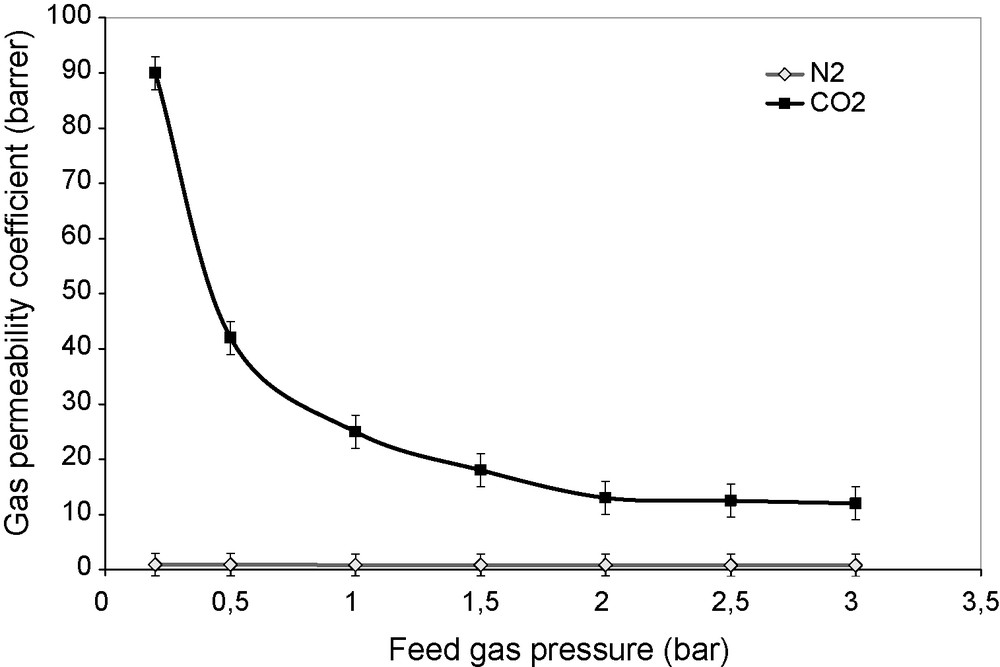
Gas permeability coefficient versus feed gas pressure for the PVA/PEI/PEG 60/20/20 membrane tested with pure CO2 and N2, at 25 °C.
1 barrer = 10−10 cm3 (STP) cm−1 s−1 cm Hg−1.
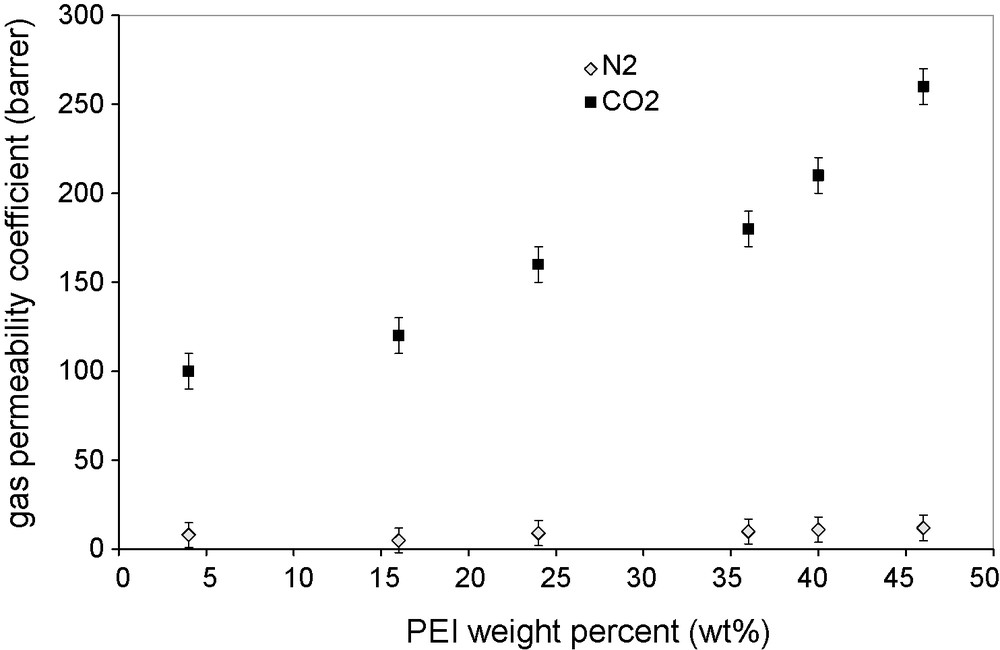
Effect of the PEI content (wt%) on CO2 and N2 permeabilities through PVA/PEI/PEG membranes. Feed gas pressure 1 bar; temperature 25 °C.
The CO2 facilitated transport through the PVA/PEI/PEG membranes should depend negligibly on the molecular weight of the polymer carrier, since the membranes made with PEI of average molecular weights of 2000 and 25,000 had similar characteristics. This indicates that the PEI chains were immobilized in the PVA matrix in the same way whatever their length, and the active amine groups effectively contribute to the facilitated transport with the same parameter values.
3.2.2 Effect of PEI content
Figs. 7 and 8 show the effect of PEI content on the permeability and the ideal selectivity of the prepared membranes at a gas pressure of 1 bar. The highest selectivity obtained, 24, largely exceeds that of a PVA membrane (Fig. 7). The selectivity initially increased with the PEI content, reached a maximum, then decreased and levelled-off with further increase in the PEI content. The increase in the permeability with the increase in the content of carrier in the membranes is quite general and is explained by the larger contribution of the facilitated transport to the total permeation. Therefore, the decrease in the facilitation effect beyond a certain PEI content can only be explained by a change in the values of the material transport parameters. Cai et al. [8] studied the change in the crystallinity of the PVA membranes made of PVA blends with polyallylamine, with the polyamine content and found that there was an inverse relationship between the PVA crystallinity and the gas permeance of the blend membranes. The situation should be the same with our membranes: the PVA crystallinity would go through a minimum at the content of maximum permeability. The compact organization of polymer crystallites prevents any sorption of the permeant molecules in their structure, and makes the molecular diffusion in the remaining amorphous phase much slower by creating impermeable obstacles on the diffusion pathways. In the case of PVA, its crystallinity was shown to have a dramatic effect on the PVA membrane permeability [23]. The PVA crystallinity effect is much smaller in the case of PVA–PEI and PVA–polyallylamine blends, because the main contribution to the total transport was from the facilitated transport via the amorphous amine carriers.
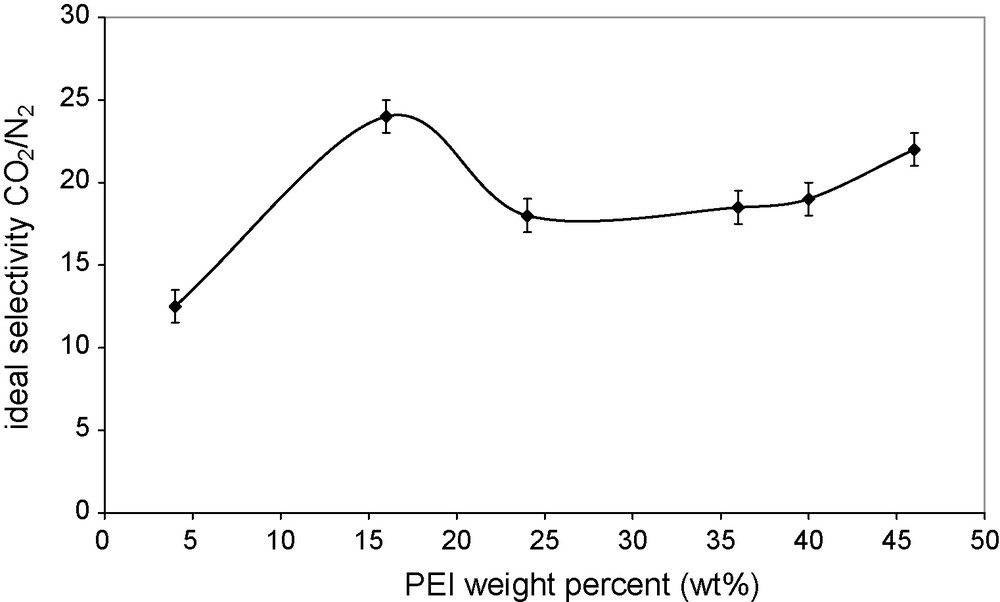
Effect of the PEI content (wt%) on CO2/N2 selectivity in PVA/PEI/PEG membranes. Feed gas pressure 1 bar; temperature 25 °C.
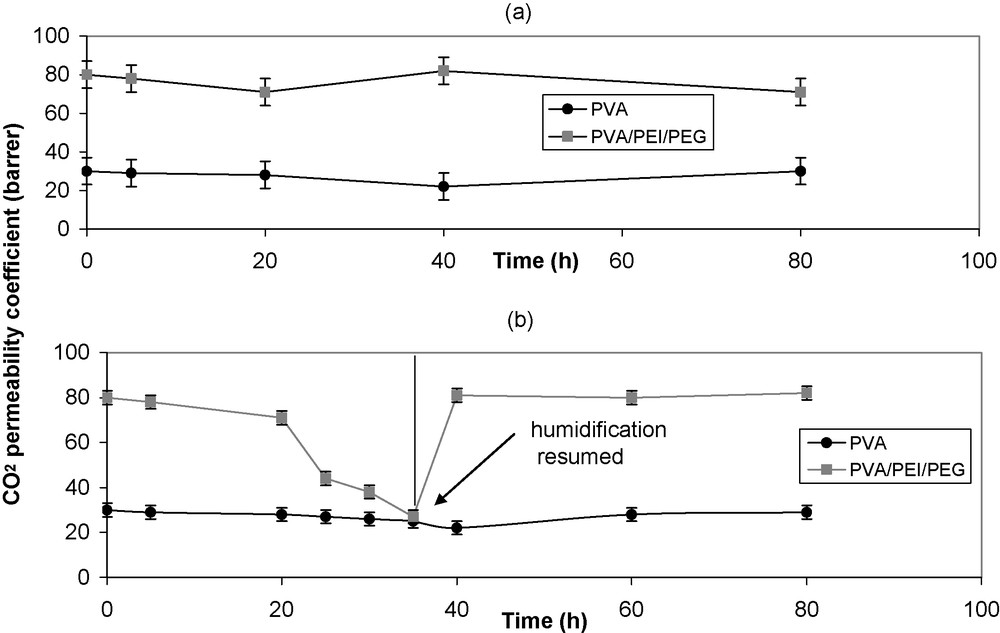
(a): Effect of long-term permeation with humidified CO2 on membrane permeability. Experimental conditions: PVA and PVA/PEI/PEG 60/20/20 membranes with a feed gas at 1 bar pressure and 25 °C; the feed gas was continuously humidified (at 20% relative humidity); (b): variation in CO2 permeability with time for the same membranes when the gas humidification was disrupted, then restored.
We chose to blend PEG with PVA and PEI in expecting a positive contribution of this polymer to the CO2 transport via the normal solution–diffusion mechanism. Intrinsically, PEG does not show extremely high performance: CO2/N2 separation factors of 48, and CO2 permeability of 12 barrers [24] due to the high crystallinity of pure PEG membranes. The CO2 permeability of PVA/PEI/PEG membranes appears to be high (better than 100 barrers for a CO2 pressure of 0,2 bar), but their CO2 selectivity low (Figs. 5 and 6) compared with other membranes. Compared with segmented copolymers like poly(ether- block- amide), their CO2 permeability is comparable [24,25], and their selectivity much lower (for CO2 with methane and nitrogen). In the membranes made of PEG segmented copolymers, the best performance would be obtained with PEG as the continuous phase. In our PVA/PEI/PEG membranes, PEG would be partially associated with PVA in its amorphous form by hydrogen bonds (as stated above), but the main part of PEG would be separated into a PEG-rich dispersed phase. The increase in the density of dispersed globules with the PEG content in the membrane supports this argument. We speculate that such phase separated PEG-rich globules in the PEI/PVA/PEG caused a fall in the selectivity of the PVA/PEI blend membrane, which was reported to be 160 for CO2/N2 under 0.065 bar of CO2 pressure [7].
The membranes with low added-PEG amounts (up to 8 wt. % of PEG) exhibited, within the experimental error limit, similar characteristics as the membrane without PEG. Apparently, a small amount of PEG did not exhibit any noticeable active function. It was noticed that membranes of intermediate PEG contents (10–15 wt. % of PEG) had unstable, thus not interpretable, characteristics. This can be due to unstable structures of the blends, that could be explained by a reorganization of chains, or a migration of PEG chains between phases at the compositions close to binodal locus in the multiphase diagram. PEG incorporation in the PVA/PEI blend did not bring about the seeked benefit, i.e., a higher membrane selectivity towards CO2 via PEG sorption selectivity.
3.2.3 Stability of the membrane
Membrane stability is an important parameter that determines the practical feasibility of the membranes. The main problem for facilitated transport membranes is their instability. To examine the stability of the PVA/PEI/PEG membranes, CO2 permeation experiments were carried out over a prolonged period of time. For comparison purposes, the stability of a pure PVA membrane was also tested. Throughout the experiments, the feed gas was humidified to retain the membrane permeability. In order to illustrate the importance of gas humidification to the membrane permeability, experiments were also performed when the humidification was discontinued.
The CO2 permeability of the blend membrane with added PEG decreased progressively but significantly when the humidification was stopped, probably due to a rather slow deswelling of the membrane in the continuous stream of dry gas. In contrast with this, the permeability jumps up rapidly to a stable level when the gas humidification is restored, because of a fast water uptake to a steady swelling level in the gas stream at constant humidity (Fig. 8b). The blend membrane without PEG exhibits a much smaller decrease in permeability upon humidity disruption. Such behavior reflects the higher hydrophilicity of the membrane with PEG. Similar results were reported for other facilitated transport membranes [26].
A much longer period of stability tests (≈ 4 weeks) was conducted, and no significant deterioration in the membrane properties was observed. The high hydrophilicity of the blend membranes and the immobilization of the PEI carrier in the blend structure by hydrogen bonds with PVA were probably the causes of the performance stability.
4 Conclusions
PVA/PEI/PEG blend membranes were prepared and studied in pure CO2 and N2 gas permeation. PVA and PEI were shown to exhibit extended hydrogen bonding that reduced the membrane crystallinity and promoted the miscibility between PVA and PEI. PEG also exhibited hydrogen-bonding interactions with PVA, but the interactions should be weaker, as its hydrogen bond acceptor (ether O) is much weaker than that of PEI (amine H). This explains the low miscibility between PVA and PEG, and a phase separation. DSC experiments evidenced a decrease in PVA crystallinity due to these hydrogen-bonding interactions.
The CO2 permeability decreased with the increase in the CO2 partial pressure, whereas the N2 permeance was nearly constant. This result showed that only CO2 was transported by the facilitated transport mechanism and also that PEI effectively worked as a carrier for CO2 transport. The CO2 and N2 permeabilities increased monotonically with increasing PEI contents in the membrane, probably because of a decrease in the PVA crystallinity with the PEI content increase. On the other hand, the selectivity showed a maximum. The highest CO2/N2 selectivity was about 24, which was higher than that of the pure PVA membrane, but much smaller than that of other facilitated transport membranes. The blend membrane was stable for at least 7 days of continuous permeation with humidified gases.