1 Introduction
In electromagnetic radiation, near-infrared (NIR) region ranges approximately from 650 to 1500 nm. There is constantly growing interest in NIR luminescence in the last decade because of its potential for bioanalytical applications and biological imaging, as well as for technological applications. The use of NIR luminescence is a promising approach for biological imaging and bioanalytical applications for three main reasons: (1) NIR photons have less interference with biological materials as they are mostly not absorbed by biological tissues [1]. Therefore, photons can penetrate deeply into tissues allowing noninvasive detection of biological molecules or events. (2) Since the autofluorescence arising from biological tissue is mainly located in the violet–blue region [2], imaging in the NIR region also provides the advantage of detection sensitivity due to improved signal to noise ratio, the background emission (“autofluorescence”) of biological material in the NIR being almost inexistent [1]. (3) An additional advantage of NIR photons for biological imaging is the limited scattering of such photons in comparison to visible photons that results in improved image resolution. As the intensity of scattered light is proportional to 1/λSP (SP = scattering power), the longer wavelength of NIR light has reduced scattering, which results in the higher resolution of the obtained image [3].
Beside the biological imaging, NIR luminescence is useful for NIR organic light-emitting diode technology [4], telecommunication where the electronic structure of lanthanide ions such as Er3+, Nd3+ and Ho3+ can be used as the active material for optical amplification of the NIR signal [5], and encrypted tags (“barcodes”) to recognize the identity of chemical or biological entity or of an object [6].
In general, the luminescence of lanthanides possesses several advantages over organic fluorophores [7], semi-conductor nanocrystals [8] and carbon nanotubes [9], such as sharp emission band, photostability and long luminescent lifetimes.
Luminescent lanthanides can have emission bands ranging from UV to NIR regions, controlled by the nature of lanthanide cations. As shown in Fig. 1, Nd3+, Er3+, Ho3+, Tm3+ and Yb3+ have NIR emission, while Tb3+ and Dy3+ have visible emission. The emission bands from Sm3+ and Eu3+ are located in both the visible and NIR regions. Since interactions between lanthanide cations and ligands are predominately electrostatic [10], the emission of lanthanide complexes appear as atom-like, sharp emission bands [11,12], and the wavelength position of these bands does not vary significantly with change of ligand or experimental conditions such as temperature, pressure, pH or biological environment. The bandwidths are significantly narrower than those of organic fluorophores and luminescent semiconductor nanocrystals (quantum dots) [13]. As these emission bands do not overlap significantly, it is possible to monitor the emission of several different lanthanide cations during the same experiment for multiplex detection.

Normalized emission spectra of luminescent lanthanide complexes in solution, illustrating the sharp emission bands and minimal overlap of lanthanide luminescence. Adapted from [11] and [12].
Since the energy is absorbed by a chromophore (or “antenna”, see below) and the emission originates from the lanthanide cations, there is a large energy gap between the absorption and emission bands of lanthanide complexes, minimizing luminescence reabsorption and corresponding quantification artifacts. Due to their electronic properties, the luminescence of the lanthanide cations can be spectrally discriminated from other signals, allowing enhanced detection sensitivity in complex mixtures such as biological media.
Most lanthanide compounds are photostable [14]. Trivalent electron-deficient lanthanide cations stabilize the excited states of bound organic sensitizers, preventing irreversible photoreactions when the complex is irradiated. Therefore, protection from light is not required, yielding a long shelf life and easy manipulation in ambient laboratory conditions. Most importantly, this photostability allows for long exposure times and repeated experiments.
The luminescence lifetimes of lanthanide cations are in the range of micro- to milliseconds [15], which is much longer than the pico- to nanosecond lifetimes that are typical for fluorescent organic molecules and proteins. The long luminescence lifetimes allow simple and accurate discrimination of the lanthanide complex signal from autofluorescence (background fluorescence) through time-resolved measurements, providing enhanced detection sensitivity [16].
Since f → f transitions are forbidden by the Laporte rule, free lanthanide cations have low extinction coefficients and can therefore not be excited directly with a good efficiency [10]. As a result, the number of emitted photons will be small which will result in low detection sensitivity. A strategy to overcome this limitation that has been initially disclosed by Weissman in 1942 [17] consists in locating a sensitizer at a controlled distance to the lanthanide cation. This sensitizer must fulfill the double function of absorbing as many photons as possible and to convert the resulting energy to the lanthanide metal ion. This combination of processes is known under the name of “antenna effect” (Fig. 2).

Schematic representation of the antenna effect.
Three possible mechanisms have been proposed for the energy transfer process from the donating electronic states of the sensitizer to the accepting energy levels of the metal center. (1) The Förster mechanism is a dipole–dipole type of interaction between the donor and acceptor. This mechanism requires spectral overlap between absorption of the acceptor and emission of the donor. This mechanism takes place through space; and the efficiency is dependent on r−6, where r is the distance between the donor and acceptor [18]. (2) The Dexter mechanism is a concerted electron-exchange mechanism. It requires electron exchange between the donor and acceptor, therefore orbital overlap between the donor and acceptor is necessary. The efficiency is proportional to e−r, where r is the distance between the donor and acceptor [19]. (3) Electron transfer mechanisms is a process which is dependent upon orbital overlap and the oxidation and reduction potentials of the donor and metal ion. It is limited to NIR emitting Yb3+ that can be readily reduced (E0(YbIII/YbII) = −1.05 V) [20,21]. Through this mechanism, even non-fluorescent electron rich chromophores can still sensitize Yb3+ emission [21(d)].
In order to obtain adequate/sufficient emission intensity arising from lanthanide ions, the sensitizers–antenna(e) must fulfill several requirements: (1) the sensitizers need to remain at sufficiently close distance to the lanthanide cations for a good energy transfer to occur. It implies that the compounds should not dissociate easily or should dissociate at a slower pace in comparison to the experiment to be done. (2) It is usually hypothesized that in order to maximize the efficiency of the energy transfer, the energy of the donating levels of the sensitizers must match the accepting energy level of the lanthanide ions. It is considered that in most of the cases, efficient donating levels are triplet states located on sensitizers. (3) In many lanthanide systems, overtones of –OH, –NH and –CH vibrations can provide a very efficient route of deactivation to the excited states of the lanthanide cations [15]. A strategy to prevent such a situation from happening is to shield the lanthanide cations from their environments. Such protection of the lanthanide cations is considered as being especially important when the luminescent lanthanide compound will be used in aqueous solution. In addition, in order to be easily adapted for a broad range of applications, the sensitizer should be easy to modify in order to control chemical, physical and/or photophysical properties of the resulting luminescent lanthanide compound.
The field of development of sensitizers for NIR emitting lanthanide cations is currently booming. A large number of examples can be found in the literature. Several sensitizers or antennae have been described for the sensitization of NIR emitting lanthanide cations [21–57]. Nevertheless, the number of antennae for NIR emitting lanthanides is still limited and deserved more investigations as only a few lanthanide compounds match the requirements for practical applications in biological media [16]. One of the strongest limitations for applications remains the ability to emit a sufficiently large number of photons per unit volume that will allow obtaining good detection sensitivity. Typically, the actual complexes formed with NIR emitting lanthanide cations have low quantum yields in comparison to visible emitting lanthanide compounds limiting the number of emitted photons per discrete molecule.
In this review, we present and discuss several strategies and chemical systems that have been created and studied in our research group in order to develop novel antennae for NIR luminescent lanthanide cations. The strategies divide themselves in two main categories: (1) the formation of monometallic lanthanide complexes using sensitizers based on derivatives of salophen, tropolonate, azulene and pyridine; and (2) the formation of polymetallic lanthanide compounds using several different strategies such as incorporation of luminescent lanthanides in nanocrystals, metal-organic frameworks (MOFs) containing lanthanide cations and polymetallic dendrimer complexes. The polymetallic systems are promising for practical applications, since in these systems, the amount of lanthanide emitters per unit of volume and the overall absorption of each discrete molecule can be significantly increased. This advantage is appealing to enhance detection sensitivity.
2 Monometallic complex systems
2.1 Complexes formed with salophen derivatives
We have developed a series of ligands designed for the coordination and sensitization of near-infrared emitting Nd3+ by modifying salophen Schiff base with different numbers and locations of bromide substituents (Fig. 3) [58].

Structure of Nd3+ complexes of salophen derivatives.
The nature of the Nd3+ complexes in solution was determined to be [ML2]−. Spectrophotometric titrations indicate that the different substituents do not affect significantly the nature of the formed species. The structures of the corresponding complexes have been determined in solid phase from X-ray diffraction experiments. The stoichiometries and structures in solid state appear to be different from those observed in solution. One complex showed [ML2]− stoichiometry, while the other formed M2L3 complex (Fig. 4). We have identified that the structures in solid state can be partially controlled by crystallization conditions.

ORTEP representations of the molecular structure of (a) [Nd(L4)2]− with Et3NH+ as counter cation and (b) Nd2(L1)3·MeOH (50% probability ellipsoids, H atoms have been omitted for clarity) [58]. Copyright Wiley-VCH Verlag GmbH & Co. KGaA. Reproduced with permission.
These ligands have the ability to sensitize Nd3+ through intramolecular energy transfer from the ligand to the metal ion. The absorption, excitation and emission spectra of [Nd(L1)2]− are shown in Fig. 5 as a representative example. The quantum yield values of these complexes are ranged between 1.18 × 10−3 and 2.01 × 10−3, which are comparable to that of other Nd3+ complex in DMSO [12]. We could notice that the presence of bromo groups on the salophen ligands enhances the efficiency of the energy transfer from the sensitizer moiety to the Nd3+: the greater the numbers of bromo groups, the higher the quantum yield values. This result can be attributed to an increase in the population of the triplet state due to the heavy atom effect generated by the presence of bromo groups.

Normalized absorption (solid), excitation (dotted; λem = 1058 nm), and emission (dashed; λex = 395 nm) spectra of Na+[Nd(L1)2]− complex (4.64 × 10−5 M in DMSO at 298 K). [58]. Copyright Wiley-VCH Verlag GmbH & Co. KGaA. Reproduced with permission.
This type of systems is appealing because of the possibility to create a large number of ligand-lanthanide sensitizers, where the electronic structure of these sensitizers is modified by the presence of the substituents on the sensitizer. This is an appealing approach to develop compounds that will be interesting for the increase of the rationalization between the structure of the lanthanide cations and the properties of the resulting NIR emitting lanthanide compounds.
2.2 Complexes formed with tropolonate
We also have successfully demonstrated that the chromophoric moiety tropolonate can coordinate and sensitize several lanthanide cations emitting in the NIR domain. Tropolonate is a seven-member aromatic ligand, which can bind metal ions in bidentate mode (Fig. 6). We have structurally characterized a large number of structures of complexes resulting from the reaction of this chromophore with lanthanide cations (Tb3+, Dy3+, Ho3+, Er3+, Tm3+, Yb3+ and Lu3+). In all cases, we have identified that the complexes with a formula of [Ln(Trop)4]− (Trop = C7H5O2; tropolonate) were formed by the reaction of four deprotonated tropolonate ligands with one equivalent of lanthanide cation [12,59].

Structure of tropolone and coordination of tropolonate with lanthanides.
The crystal of several lanthanide tropolonate complexes (Ln3+ = Tb3+, Dy3+, Ho3+, Er3+, Tm3+, Yb3+, and Lu3+) have been isolated and systematically analyzed by X-ray diffraction and the results have been compared. The analysis of the structures in the solid state reveals that the coordination geometries around the lanthanides are virtually similar for all the cations that have been analyzed. With the exception of the Tb3+ complex, all the crystal structures are isomorphous. The structure of the Yb3+ complex is shown in Fig. 7(a) as a representative example. Each lanthanide cation is coordinated by four bidentate tropolonate ligands, and the resulting coordination number is 8. The average Ln–O bond length in each of these complexes ranges from 2.31 to 2.37 Å, steadily decreasing from Tb3+ to Lu3+ (Fig. 7(b)), which can be explained by the decrease in the respective effective ionic radii of the central lanthanide ion (1.04 Å for Tb3+ and 0.977 Å for Lu3+, values obtained using Shannon [60] calculations for a coordination number of eight).

(a) ORTEP representations of the molecular structure of K[Yb(Trop)4]·DMF (50% probability ellipsoids, H atoms have been omitted for clarity); (b) Ln–O bond length in K[Yb(Trop)4]·DMF versus the reciprocal of the effective ionic radii. Reprinted with permission from [59]. Copyright 2007 American Chemical Society.
We have identified that the tropolonate chromophoric moiety is efficient in sensitizing five different NIR emitting lanthanide cations: Nd3+, Er3+, Ho3+, Tm3+, and Yb3+ in solution (Fig. 8). Quantum yields of the complexes formed with Yb3+ (1.9 × 10−2 in DMSO) are comparable to the highest reported quantum yield values for other lanthanide complexes that emit in the NIR domain in organic solvents [21(e),29,32,49]. This result is a breakthrough as the chromophore is able to sensitize five different lanthanide cations with a unique electronic structure. In the future, this system will be interesting for the fundamental study of the relationship between electronic structure and corresponding luminescence properties.
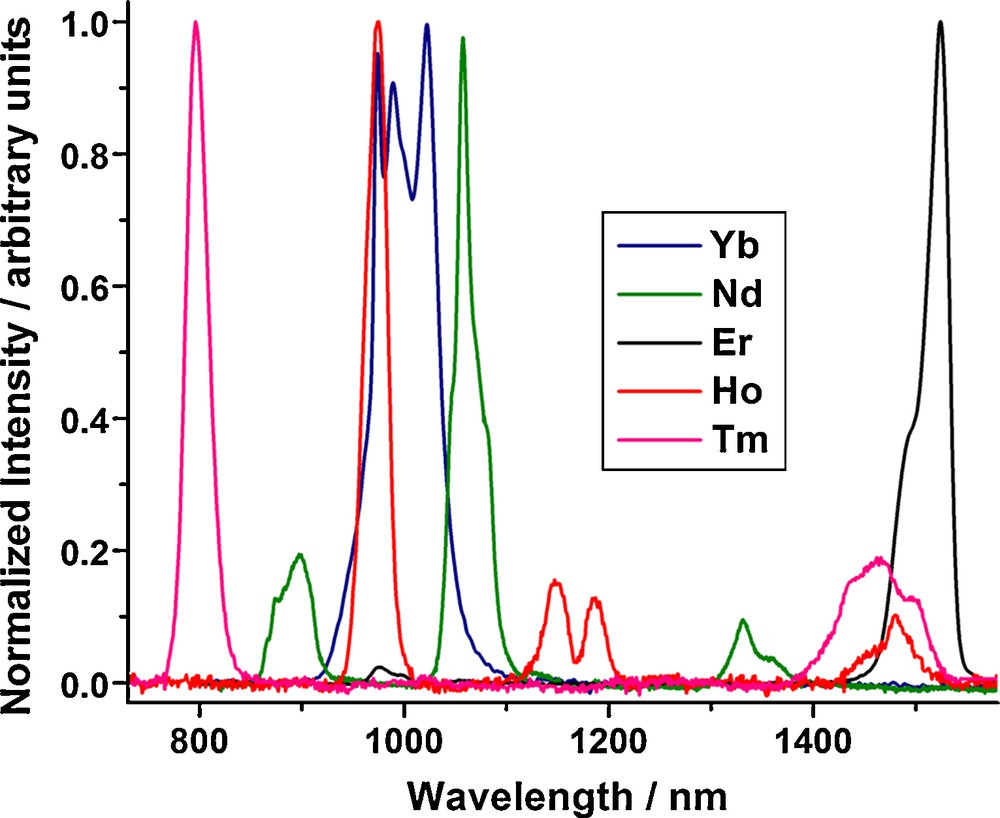
Normalized NIR emission spectra of [Ln(Trop)4]− (Ln = Yb, Nd, Er, Ho and Tm; 10−5 M in DMSO at 298 K; λex = 340 nm). [12]. Copyright Wiley-VCH Verlag GmbH & Co. KGaA. Reproduced with permission.
We identified that, for each of the lanthanide studied, the cation adopt a coordination number of nine in solution. Four tropolonate units provide eight coordination and one coordination site is occupied by a solvent molecule. The presence of a molecule of solvent induces a nonradiative deactivation of the luminescence of the lanthanide cation, especially when the solvent is water. As a quantitative result, the quantum yield value of the [Yb(L)4]− complex recorded in water is almost two orders of magnitude smaller that the value observed in DMSO. This limitation is expected to be removed in the future by attaching the four tropolonate sensitizing moieties to a common backbone which should improve the protection of the lanthanide cation against nonradiative deactivation. It is surprising to observe that the coordination motif around the lanthanide cations for the different complexes is fairly different in solution and in the solid state. This observation suggests a word of caution when data obtained from X-ray crystallography are used to rationalize the photophysical properties of lanthanide complexes. As these cations can adopt a broad variety of coordination geometries [61], a small change in this parameter can have a drastic effect of the structures of the resulting compounds.
2.3 Complexes formed with an azulene derivative
Another novel sensitizer that has been created and tested in our group is the diethyl 2-hydroxyazulene-1,3-dicarboxylate. It is an azulene derivative and shares some structural similarity with tropolonate. This ligand (HAz) can be synthesized from tropolone. Several different [Ln(Az)4]− complexes were formed with different NIR emitting lanthanide cations (Yb3+, Nd3+, Er3+, and Tm3+) coordinating in bidentate mode (Fig. 9) [62].

Structure of diethyl 2-hydroxyazulene-1,3-dicarboxylate (HAz) and proposed coordination mode of one Az− with one Ln3+.
Because of the wide conjugation in this chromophore, the triplet state of this molecule is located at significantly lower energy (13,600 cm−1) in comparison to tropolonate (17,200 cm−1). It has been hypothesized to provide a better match of energy with the accepting levels of several NIR emitting lanthanide cations. This ligand-chromophore system provides an efficient sensitization for four different NIR emitting lanthanide cations: Yb3+, Nd3+, Er3+ and Tm3+ (Fig. 10).

Normalized excitation (dashed) and emission spectra (solid) of four [Ln(Az)4]− complexes (Ln = Yb, Nd, Er and Tm; 10−5 M in CH3CN at 298 K, λex = 380 nm). [62]. Copyright Wiley-VCH Verlag GmbH & Co. KGaA. Reproduced with permission.
In order to quantify the efficiency of the sensitizer for lanthanide energy transfer and the protection of the lanthanide cation against nonradiative deactivations, the quantum yields of these complexes were recorded in solution. It was exciting to observe that the values obtained for the Nd3+ and Yb3+ complexes (3.7 × 10−3 and 3.2 × 10−2 respectively, both in CH3CN) are among the highest values reported for NIR emitting lanthanide complexes in solution. We attribute this good result to a combination of efficient ligand-to-lanthanide energy transfer and good protection of the lanthanide cation against nonradiative deactivations. The fact that the azulene-based ligand can sensitize not only Yb3+, Nd3+ and Er3+, but also Tm3+ indicates that it is a promising sensitizer for NIR lanthanide ions, potentially suitable for biological imaging. The simultaneous emission of several lanthanide signals is appealing for multiplex assays where several analytes can be detected during the same experiment. It is worth noting the sensitization of Tm3+, since one of its emission wavelength is particularly interesting for biological imaging as the emission around 790 nm corresponds to a minimum of absorption by tissues and biological fluids, an advantage appreciated for noninvasive optical imagery. These results validate the hypothesis that sensitizers with triplet states located at lower energy are well suited for NIR emitting lanthanide cations. The four bidentate ligands coordinated to the central lanthanide cation in the [ML4]− complex provide good protection against nonradiative deactivations, which is an improvement in comparison to the complexes formed with the tropolonate ligands.
2.4 Complexes formed with a pyridine-based ligands for bimodal MRI and NIR luminescence detection
In collaboration with the teams of Dr. Éva Tóth (centre de biophysique moléculaire, CNRS, Orléans, France) and Dr. Franck Suzenet (institut de chimie organique et analytique, université d’Orléans, France), we have created a platform for the creation of novel Ln3+ complexes that can act as bimodal imagining reagents, combining both optical (luminescence) and MRI (contrast agents) activities. Combining both types of imaging ability on a common molecule is attractive, as each of them possesses complementary properties. More specifically, optical imaging based on luminescence is attractive due to its high detection sensitivity. In comparison to MRI detection based on contrast agents, luminescence imaging has a lower resolution (even though the NIR photons allow obtaining a better resolution in comparison to those obtained with visible photons) [63]. For the imaging of the interior of biological systems, the depth of penetration of photons depends on the absorption and emission wavelengths of the lanthanide compounds. NIR photons are attractive as they allow the beam of photons to cross several centimeters of tissues. MRI imagery based on contrast agents has the advantage of the high resolution of the image and no limitation in crossing tissues. The limitation of MRI imagery lies in its lower sensitivity. Therefore, the detection concentrations involved in the applications of these systems are significantly different and complementary. The field of application itself can be different: imagery of entire biological systems with MRI and cellular imagery with NIR luminescence. It is attractive to combine both activities on the same molecule as the development of such a complex would require a unique characterization of the behavior of the lanthanide complexes in solution and of its biological distribution (biodistribution). The results obtained from one imaging technique will be useful to validate the lanthanide complex system to be used with the other technique. As lanthanide cations have very similar chemical reactivities, it is easy to replace a lanthanide cation such as Gd3+ that operates as MRI contrast agent by luminescent lanthanide cations such as Nd3+, Yb3+, Ho3+ or Tm3+.
The system that has been created can be used as a versatile scaffold where MRI and NIR luminescence requirements are simultaneously satisfied using a common ligand structure (Fig. 11) [64]. The analysis of the behavior of the complexes formed between one equivalent of ligand and one equivalent of lanthanide cation in solution indicate that they are remarkably inert, related to the rigidity of the pyridyl skeleton. Such inertness is an important advantage for the use of these complexes as imaging agents.

Structure of pyridine-based ligands for bimodal Ln3+ complexes.
We have observed that our pyridinic sensitizing moiety is a suitable antenna for Nd3+ (Fig. 12). More interestingly, we have demonstrated that even bishydrated Nd3+ complexes can emit a significant amount of NIR photons in aqueous solution as the pyridyl derivative unit acting as the antenna.

Normalized excitation (left; λem = 1065 nm) and emission (right; λex = 267 nm) spectra of the NdL complex formed with ligand R = H (5.1 × 10−4 M in HEPES buffer 0.01 M, pH = 7.02, ionic strength KCl 0.01 M). [64]. Reproduced by permission of The Royal Society of Chemistry.
These complexes have a quantum yield (9.7 × 10−5 for the Nd3+ complex in H2O) in the same range as those of the most optimized chelates described so far in the literature [26]. The analysis of the photophysical properties of the Nd3+ complexes formed with the ligands of this family indicate that the pyridyl moiety is a prime candidate for the development of bimodal MRI/NIR imaging probes. In the perspective of biological applications, we are currently modifying the ligand structure to displace the excitation wavelength towards lower energies where tissues and blood have lower absorbance to: (1) prevent damages to the biological samples; and (2) allow deeper tissue penetration of the photons for noninvasive optical diagnostics. The lowering of the energies of the triplet states is also expected to improve the sensitizer-to-lanthanide energy transfer, as demonstrated from our previous work based on azulene [62]. Due to the design of the platform, these structural modifications of the ligand, however, are not expected to influence the bishydrated nature and kinetic inertness of the complexes.
This prototype lanthanide complex is the first report of a lanthanide-based bimodal agent that combines MRI activity with NIR luminescence. We have demonstrated for the first time with this system that the presence of two H2O molecules bound to the Ln3+, beneficial for MRI applications of the Gd3+ analogue, is not an absolute limitation for the development of NIR luminescent agents; there exist feasible routes towards Ln3+-based, bimodal probes suitable for both MRI and NIR optical imaging.
3 Polymetallic complex system
Due to their relatively low quantum yields, most monometallic complexes emitting NIR luminescence have the limitation that the number of emitted photons is not sufficient to allow their use for a broad range of applications, as this low number of photons results in the limited detection sensitivity. This limitation should be overcome in the future by a better control of the parameters that govern intramolecular energy transfer and by improving the protection of lanthanide cations from nonradiative deactivation. However, in order to immediately take advantage of the properties of NIR emitting lanthanide complexes for practical applications, we have decided to explore a novel strategy where the number of photons per unit of molecular volume is maximized by the creation of polymetallic lanthanide emitters having very large absorption [65]. Since the luminescence intensity of a lanthanide compound is proportional to: (1) the quantum yield that itself depends on the efficiency of intramolecular energy transfer and the protection of the lanthanide cation; (2) the number of lanthanide cations per unit volume; and (3) the molar absorptivity of the compound as the more photons get absorbed, the more photons will be emitted. Therefore, polymetallic compounds with a large number of sensitizers and lanthanide cations are expected to emit a large number of photons per unit volume which will provide better detection sensitivity in practical applications.
3.1 Polymetallic dendrimer complexes
Dendrimers are well-defined, highly branched nanoscale molecules. Dendrimers are able to provide a versatile framework to create polymetallic lanthanide complexes by incorporating a larger number of sensitizers and lanthanide cations in a well-defined volume. We have designed polymetallic dendrimer-lanthanide complexes based on poly(amidoamine) (PAMAM) [66] by attaching sensitizers on the terminal branches and coordinating Ln3+ to the amide oxygen atoms located in the interior of the dendrimer (Fig. 13(a)). This synthetic design has the advantage to allow the use of sensitizing units that are not directly bound to luminescent lanthanide cations, a different situation in comparison to the system that we have developed with small molecules where the chromophoric unit is usually directly bound to the lanthanide cation, resulting in the requirement of the presence of a binding group on the chromophore. As a result, this strategy allows using a much broader choice of lanthanide sensitizers. On the fundamental point of view, these systems are interesting as the chromophore is sufficiently remote from the lanthanide cation to prevent any other type of energy transfer than Förster [18]. Such systems can then be a platform to study the chromophore to lanthanide energy transfer.

(a) Schematic structure of a dendrimer-lanthanide complex based on a generation-3 PAMAM dendrimer; (b) Polymetallic complex formed by reaction of a generation-3 PAMAM dendrimer whose branches have been functionalized with 32 2,3-naphthalimide groups with eight Eu3+ cations.
To establish proof of the principle of the validity of this conceptual approach, we have synthesized a ligand based on a generation-3 PAMAM dendrimer functionalized with 32 2,3-naphthalimide chromophoric groups on their end branches (Fig. 13(b)) [67]. The choice of the 2,3-naphthalamide group as sensitizer was based on the high population of its triplet state due to the particularly efficient intersystem crossing [68]. With this ligand system, we have demonstrated that we can identify the formation of well-defined polymetallic Eu3+ complexes using luminescence titrations. Eu3+ was chosen since it emits in the visible and in the NIR. The formation of a species corresponding to a dendrimer:lanthanide ratio of 1:8 was determined on the criteria of the strongest Eu3+ emission signal per lanthanide cation as a result of the most efficient energy transfer and most efficient protection of the lanthanide cation against nonradiative deactivations. Eu3+ possesses transitions that are located in the visible and in the NIR range (Fig. 14(a)). It took at least seven days for the complete formation of the complex, suggesting kinetic inertness. Luminescence lifetimes recorded on Eu3+ signal at room temperature (1.10 ms) and 77 K (1.16 ms) indicated that all eight cations are well protected from nonradiative deactivation by the dendritic structure. The absolute quantum yield values upon excitation of the sensitizer and monitoring the Eu3+ emission of this complex is 0.06%. Despite the limited efficiency of the sensitization of Eu3+, the emission signal is fairly intense, due to the presence of a large number of lanthanide cations per unit volume, the high overall absorption due to the presence of the 32 naphthalimide groups and the good protection of lanthanide cations in the interior of the dendrimer. This light emission in solution can be easily monitored with the naked eye under irradiation with 354 nm UV light (Fig. 14(b)). This is a qualitative confirmation that the negative effect of quantum yields can be compensated by the large number of chromophores and lanthanide cations per unit of volume.

(a) Absorption (left) and emission (right; λex = 360 nm) spectra of Eu3+ complex of naphthalimide attached PAMAM dendrimer (10−6 M in DMSO at 298 K); (b) solution of Eu3+ complex of naphthalimide attached PAMAM dendrimer (8.7 × 10−5 M in DMSO at 298 K) irradiated with excitation light at 354 nm. Reprinted with permission from [67]. Copyright 2004 American Chemical Society.
We have demonstrated that the design of this dendrimer system allows easy modifications of complexes using a broader choice of sensitizers and lanthanides. Since the chromophoric groups do not need to possess atoms or groups for lanthanide coordination, a wider variety of sensitizers–lanthanides combinations can be chosen for the complexes, including NIR luminescent lanthanides and their sensitizers. As an example of this flexibility, we have recently created a solid-state system for the sensing of oxygen by coating carbon nanotubes with dendrimers that possess a different lanthanide sensitizer: a derivative of the 1,8-naphthalimide group. In comparison to the previously described derivative of 2,3-naphthalimide, the electronic state of this sensitizer has a triplet state which is located lower in energy, a desirable property to promote interaction between the sensing part and the molecules of oxygen [69]. Another originality of this new system is that it offers a way to analyze the electronic structure of the dendrimer-lanthanide complex. From the point of view of applications, it is the basis for solid-state sensor using luminescence and/or conductance detection.
In another direction, we are currently working on several purely NIR emitting dendrimer complexes using this versatile synthetic strategy.
3.2 Tropolonate ligands for the sensitization of NIR polymetallic Ln3+-doped NaYF4 nanocrystals
As another strategy to generate polymetallic NIR luminescent compounds, we have created and analyzed lanthanide-doped nanocrystals. With this strategy, as most of the lanthanide cations are doped inside of the nanocrystal, they are well protected from the environment, which is especially appealing for NIR emitting lanthanides that are easily quenched due to the small energy gap between their excited and ground states. In order to sensitize lanthanides, several molecules of organic chromophore, tropolonate, were attached on the surface of the Nd3+ or Yb3+-doped NaYF4 nanocrystal (Fig. 15) [70]. Tropolonate was chosen as sensitizer to achieve this goal as it has been previously tested for the sensitization of NIR emitting cation in molecular complexes (see above) [12,59].
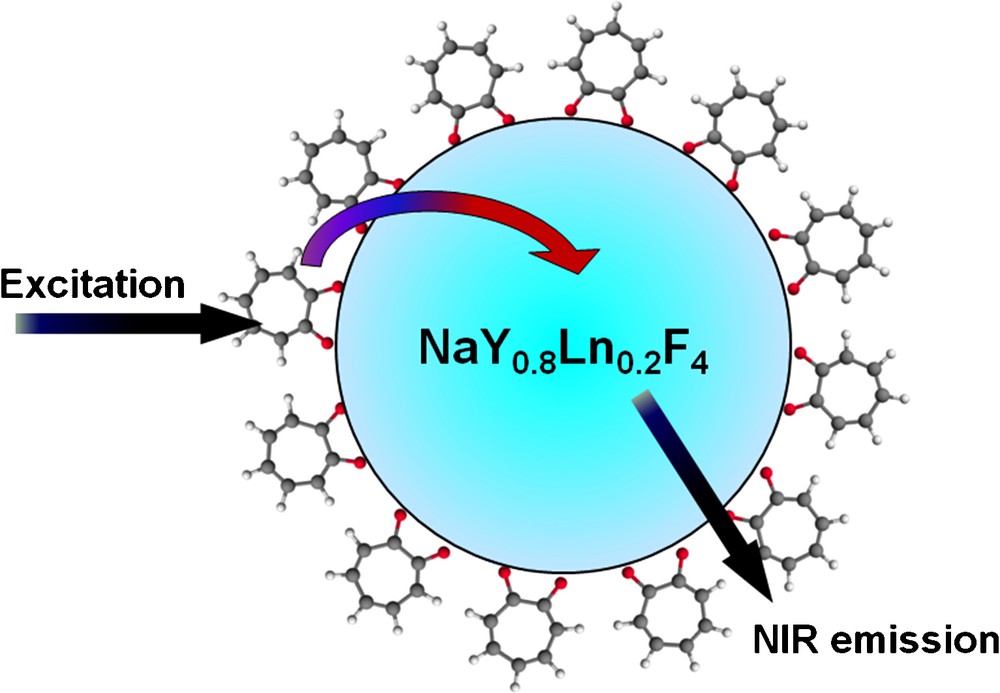
Schematic illustration of tropolonate capped Ln3+-doped NaYF4 nanocrystals (Ln3+ = Nd3+ or Yb3+). Reprinted with permission from [70]. Copyright 2007 American Chemical Society.
Combining protection against nonradiative deactivations provided by the inorganic matrix of NaYF4 nanocrystals with sensitization from tropolonate ligands capped on their surface, the lanthanide cation centered luminescence was observed through the ligand excitation and demonstrates that tropolonate ligands can provide an antenna effect even when not directly bound to the lanthanide cations (Fig. 16).
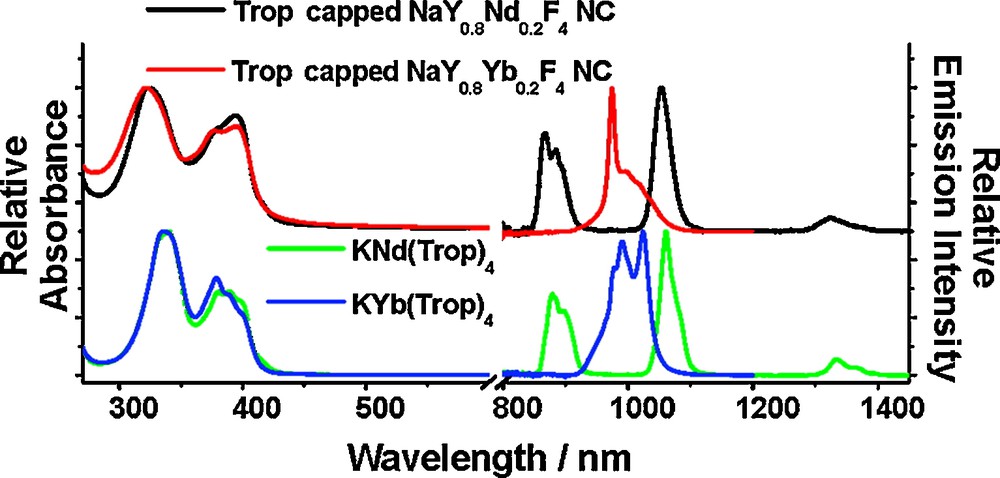
Normalized absorption (left) and emission (right; λex = 340 nm) spectra of KLn(Trop)4 complex (bottom; 10−4 M in DMSO) and Trop− capped nanocrystals (top; ca. 1 g L−1 in DMSO). Reprinted with permission from [70]. Copyright 2007 American Chemical Society.
Significantly longer luminescence lifetimes (5–10 fold) were observed for the Trop-capped Yb3+/Nd3+ doped nanocrystals (the longest lifetimes are 68 μs and 12.6 μs for Yb3+- and Nd3+-doped nanocrystals, respectively) in comparison to the corresponding molecular complex [Ln(Trop)4]− (12.43 μs and 1.10 μs for [Yb(Trop)4]− and [Nd(Trop)4]−, respectively) [12]. The extended lanthanide luminescence lifetimes indicate the success of this strategy aimed at protecting the lanthanide cations against nonradiative deactivations. This protection and sensitization strategy can be potentially extended to a broader range of antenna for the optimized energy transfer. The effect of the number of tropolonate ligands on the surface and lanthanides and the relationship to the overall quantum yields of the compounds will be rationalized with further studies.
3.3 Metal-organic frameworks for NIR Emitting polymetallic lanthanide complexes
In collaboration with Professor Nathaniel L. Rosi (Department of Chemistry, University of Pittsburgh), we have tested an approach based on the use of metal-organic frameworks (MOFs) as a strategy to coordinate and sensitize NIR emitting lanthanide cations, through the formation of systems with well-defined structures. This approach is another exciting example of the strategy for the controlled creation of compounds possessing a large number of lanthanide emitters and chromophores to maximize the number of photons emitted per unit of volume. The high degree of organization and rigidity of this material is appealing for the creation of photonic materials with controlled properties, as the lanthanide cations are precisely located in a tridimensional space. These locations can be easily determined by X-ray analysis. An exciting potential application of such materials is their use as sensors due to the presence of internal cavities that have high reactive surfaces [71].
In these materials, the cations are incorporated into MOFs as the metal component of networks formed with chromophoric organic groups. The organic groups sensitize lanthanide luminescence, while the MOF framework protects lanthanide cations from quenching through nonradiative deactivations. These rigid architectures provide the ability to control the environment around the lanthanide cations, achieving specific coordination geometries for the cations and also allowing control over the organic components’ arrangement in space, enabling interactions between chromophoric groups that possess lower excitation energy ranges. Two unique MOF structures have been formed with Yb3+ and a phenylene moiety, 4,4′-[(2,5-dimethoxy-1,4-phenylene)di-2,1-ethenediyl]bis-benzoate (PVDC), Yb-PVDC-1 and Yb-PVDC-2 (Fig. 17(a)) [72].

(a) Structural depiction of Yb-PVDC-2 MOF; (b) excitation (dotted; λem = 980 nm) and emission (solid; λex = 500 nm) spectra of Yb-PVDC-2 MOF, and excitation spectrum of corresponding molecular complex in DMSO solution (dashed; λem = 980 nm). MOF as crystalline solid under chloroform. [72]. Reproduced by permission of The Royal Society of Chemistry.
The MOFs display Yb3+ centered luminescence with red-shifted excitation bands in comparison to the corresponding molecular complex (Fig. 17(b)). They have long luminescence lifetimes (29 and 22 μs for Yb-PVDC-1 and Yb-PVDC-2, respectively), and quantum yields (3.3 × 10−3 and 1.8 × 10−2 for Yb-PVDC-1 and Yb-PVDC-2, respectively) that are among the highest reported values for NIR emitting lanthanide compounds. The long luminescence lifetimes confirm that the NIR emitting actions possess good protection of the lanthanide cations against nonradiative deactivations.
The lanthanide cations do not appear to be strongly quenched by the vibrations of the solvent or of the chromophore. As an application for this technology, we have recently developed a new conceptual approach for creating luminescent barcoded systems based on MOFs, which contain multiple NIR emitting lanthanides. Barcoded materials are encrypted labels or tags to recognize the identity of materials or objects. There are several required properties of barcoded luminescent materials: they should be robust, available in large scale, and their synthesis should be straightforward, highly reproducible, and configurable to provide numerous possible encoding combinations. In addition, its spectral signature should be easily and rapidly acquired and unambiguously interpreted with inexpensive and portable equipment. In a luminescent barcoded system, an item can be labeled with a compound containing several luminescent components in a unique and controlled ratio.
We have adapted previously studied Yb-PVDC-1 to yield barcoded frameworks containing both ytterbium and erbium: ErxYb1−x-PVDC-1 MOFs. Yb3+ and Er3+ were chosen because they have distinguishable emission profiles in the NIR (Fig. 18) [6].

Yb3+ emission (980 nm) and Er3+ emission (1530 nm) spectra recorded upon 490 nm excitation, (a) normalized to the Er3+ signal; (b) normalized to the Yb3+ signal; (c) a plot of the ratio of integrated emission intensities versus their atomic ratio measured by EDS, (blue, λex = 370 nm; pink, λex = 490 nm); (d) color-coded schematic of the barcode readout. Reprinted with permission from [6]. Copyright 2009 American Chemical Society.
ErxYb1−x-PVDC-1 MOFs have huge potential to fulfill all the requirements for barcoded systems, and will be useful for several practical applications. To evaluate the possibility for such a practical application, we coated one of these MOFs in superglue (Fig. 19(a)) and then investigated its luminescence properties. Upon excitation at 490 nm, the Yb3+/Er3+ barcode was easily detected in the NIR range (Fig. 19(b)) as the presence of the superglue did not interfere with the detection of the signal.

(a) Er0.58Yb0.42-PVDC-1 sample, dried and glued to a microscope slide (an American dime coin is included for size perspective); (b) Yb3+ emission (980 nm) and Er3+ emission (1530 nm) spectrum (λex = 490 nm) of this sample. Reprinted with permission from [6]. Copyright 2009 American Chemical Society.
4 Conclusion and perspective
In this review, we summarized our efforts on the creation and investigation for novel antennae to sensitize NIR luminescent lanthanide cations. There are strong needs for NIR luminescence compounds in many fields from information technology to biological applications. In comparison to the sensitization of visible luminescent lanthanides, the sensitization of NIR luminescent lanthanides is more challenging, because the excited states of NIR luminescent lanthanides can often be easily affected by the nonradiative deactivation from the environment. The sensitizers should be well designed to transfer energy to the lanthanides efficiently and to provide adequate protection of lanthanides. We have achieved these goals to some degree with our monometallic complex systems. However, there is still room to improve the sensitizers in order to allow the use of the resulting compounds to be used in practical applications. Our approach using polymetallic lanthanide compounds with high absorptivities are promising for the use of NIR emitting lanthanide cations in a larger/broader range of applications. We have demonstrated that we can compensate a low quantum yield of lanthanide emissions with enhancing the absorption and emission by incorporating a large number of chromophoric groups and lanthanides cations. Some of these techniques can be easily applied in biological/medical imaging or industrial fields with a reasonable level of modification. We will expand our research to improve our ability to rationalize the energy transfer (mechanisms and parameters that control it) that are governing the properties of the different NIR luminescent lanthanide compounds that are described here. We hope that such work will allow us to control/improve the properties of these compounds with the ultimate goal of being able to design compounds that have predicable NIR luminescence properties. In this spirit, we will keep following a fundamental approach to create compounds that can be used for practical applications.
Acknowledgements
Funding (complete or partial) for the different projects presented in this review article was provided through the University of Pittsburgh, through the National Science Foundation (award DBI0352346), the National Energy Technology Laboratory's on-going research in clean coal technology under the RDS contract DE-AC26-04NT41817 and the National Institute of Health (award 1R21CA133553). S.P. gratefully acknowledges Le Studium (Agency for research and international hosting associate researchers in “Region Centre”), La Ligue contre le Cancer and the University of Orléans, Orléans, France, for financial support. The research efforts of many undergraduate, graduate and postdoctoral associates involved in this work are also acknowledged.