1 Introduction
Due to its major role in various biological processes, iron is one of the most vital elements [1]. To quote a few, iron complexes are involved as oxygen carrier within the body (haemoglobin, myoglobin) and are the reactive catalytic centres in several enzymes. Thus, deregulation of its metabolism can have major impacts. Abnormal iron storage, observed in genetic hemochromatosis disease, can cause liver and kidney damage [2]. Similarly, there seems to be a correlation between the increased amount of iron in the brain and cases of Huntington's, Parkinson's and Alzheimer's diseases [3]. On the other hand, deficiencies or a problem to use iron leads to anaemia [4]. Consequently, the conception of new analytical tools to accurately measure the iron concentration, in a selective and rapid manner, would be very helpful both as a diagnostic tool and as a research tool to probe iron metabolism for a better understanding. For such applications, the design of functionalized micro-electrodes is appealing as it would allow following intra- and extra-cellular ion concentrations [5]. Recently, different studies aiming at developing potentiometric iron sensors have been reported. These are mostly PVC-based membranes containing specific ionophores such as crown ether [6], EDTA derivatives [7], benzylthiocarbohydrazide [8], imidazolidine [9] or cyclam [10].
Formation of silica-based hybrid materials (either class I or class II) was largely reported, during the last 10 years, as a promising route towards new sensitive elements for the development of efficient ion sensing tools [11–14]. The main advantage of this kind of material is the possibility of truly tailoring the inorganic matrix formed using the very versatile sol-gel route [15–17]. Such chemistry allows the chemical modification of silica by either a co-condensation or a post-grafting process with organosilane reactive groups, which permit the anchoring of ionophores or molecule-selective receptors. Consequently, the domain has become very prolific and many hybrid materials have been created and designed for the electrochemical or optical detection of various species such as, for instance, DNA [18], phenol [19], trinitrotoluene [20], pesticides [21] or ionic species [22–24].
To the best of our knowledge, while the detection of cations, including some transition metal ions, was reported (for instance, Hg2+ [25] Cu2+ [26] and Cr3+ [27]), no hybrid material was reported for iron sensing. First, we studied the potentiometric response of cyclam-modified silica [28]. This study demonstrated that an optimized ionophore-modified silica matrix can allow the formation of potentiometric ion sensors (similar materials were previously reported for sensing but in an amperometric mode [29]). We report here the use of specifically designed ligands for the detection of iron (III).
The idea was to first design a supramolecular receptor incorporating a known specific tridentate iron chelator: 4-[3,5-bis(2-hydroxyphenyl)-1,2,4-triazol-1-yl]-benzoic acid called ICL670. This was recently developed as a therapeutic agent (Deferasirox, commercial designation) for the treatment of iron overload and approved in 2005 by the US Federal and Drug Administration (FDA). The macromolecular receptor was thus prepared and consists of a calix[4]arene platform onto which the chelator was grafted [30]. Association of two ICL670 chelators within the same molecule should support the octahedral complexation from the two tridentate ligands (calix-bisICL) [31]. Due to the specific chemistry of calixarenes, we were able to introduce an amino group fragment onto the calix[4]arene, in partial cone conformation, to enable the grafting process onto an organo-modified silica material (Fig. 1).

Representation of the organo-modified silica particles prepared for iron (III) sensing.
The formation of hybrid materials containing calixarene entities has been previously reported, for either the preparation of stationary phases for chromatographic applications [32,33] or for the use of the calixarene derivative as support to conceive selective caesium recovering material [34,35].
Here, we present the detailed study of the synthesis and the characterization of such type of new hybrid material specifically designed for the potentiometric detection of iron (III) in aqueous media. This new material was obtained by grafting the modified calix[4]arene (calix-bisICL) on nanosized spherical silica particles prepared using the Stöber's process (Fig. 1). We finally report on the potentiometric properties of this new sensitive material towards dissolved iron (III).
2 Experimental
2.1 Chemicals
TetraEthOxySilane (TEOS, 98%), ammonia (28%) and absolute ethanol were purchased from Acros Chemicals and used as such. Chloropropylethoxysilane, acetonitrile, TriEthanolAmine (TEA) and all the washing solvents were obtained from Aldrich and used as received. The calix[4]arene-modified with the ICL670 iron (III) chelator was synthesized following the recently reported procedure [30].
2.2 Synthesis
2.2.1 Synthesis of the silica nanoparticles (Si-Nps)
Silica nanoparticles (Si-Nps) were obtained by using the Stöber's process [36]. The synthesis consists of a temperature-controlled hydrolysis-condensation of TEOS in alcoholic media. Briefly, to a mixture of 100 mL of absolute ethanol and 7.5 mL of ammonia (28%) stirred at 400 rpm, we added 3 mL of TEOS at 70 °C. After 15 min stirring, a translucent colloidal suspension is formed and maintained under stirring for a further 12 h to complete the siliceous precursor hydrolysis; the nanoparticles were recovered by centrifugation (12,000 rpm) and abundantly washed with absolute ethanol and distilled water.
2.2.2 Synthesis of the chloropropyl-silica nanoparticles (CP-Si-Nps)
Typically, 1 mL of chloropropyltriethoxysilane was added to a suspension of 1 g of silica in dry toluene (30 mL). The resulting mixture was heated at 90 °C for 16 hours under magnetic stirring. Then, the reactive nanoparticles were recovered by centrifugation (12,000 rpm) and washed successively with dry toluene and ethanol. The as-synthesized material was finally dried under vacuum at 90 °C for 5 h.
2.2.3 Determination of the chloropropyl content in chloropropyl-silica nanoparticles (CP-Si-Nps)
To determine the amount of chloropropyl linkers grafted onto the silica surface, we first carried out a quantitative chemical transformation of this function (checked by EDX) into a nitrogen-containing linker, which can be quantified by elemental analysis. Such a method allows the quantification even in the presence of residual ethoxy groups coming from either the native Si-Nps or from the incomplete hydrolysis of the organosilane. Typically, we performed the reaction of the CP-Si-Nps with a large excess of sodium azide in the presence of a quaternary salt (TetraButylAmmoniumBromide) to entirely transform the chloromethylene entities into azidomethylene. After this step and after verifying the total absence of residual chlorine by EDX, the nitrogen content of the azidopropyl fragment (visible by FTIR at 2100 cm–1), was determined using elemental analysis. This way we obtained 0.45% of nitrogen by gram of material indicating 0.11 mmol.g–1 of azide and by deduction 0.11 mmol of chlorine per gram of CP-Si-Nps.
2.2.4 Synthesis of the calixarene-modified silica nanoparticles (calix-Si-Nps)
The immobilization of the calixarene platform (calix-bisICL) on the chloropropyl-silica nanoparticles (calix-Si-Nps) was carried out in dry acetonitrile in the presence of TriEthanolAmine (TEA). Typically, one equivalent of functionalized calix[4]arene (per chloropropyl linker) and TEA were added to a suspension of CP-Si-Nps in 20 mL of acetonitrile. The mixture was heated under reflux and magnetically stirred for 16 h; the sample was recovered by centrifugation at 12,000 rpm. Finally, after three washing cycles with dichloromethane, ethanol and diethylether, the calix-Si-Nps were dried under vacuum at 100 °C for 5 h.
2.3 Characterization
The particle morphologies were investigated using an Environmental Scanning Electron Microscope (ESEM) FEI Quanta 200 FEG equipped with an EDS analysis system (Oxford Link Isis). FTIR spectra, in a diffuse reflectance mode (DRIFT), were recorded at room temperature with a Nicolet AVATAR 370 DTGS spectrometer provided by Thermo Electron Corporation. The spectra were acquired at room temperature from 400 to 4000 cm–1 with a resolution of 2 cm–1.
Thermo-Gravimetric Analyses (TGA) were performed on a Netzsch thermal analyzer STA 449 C Jupiter equipped with a Differential analysis microbalance. The samples (10 to 15 mg) were heated, in an alumina crucible, under air from RT to 1000 °C with a heating rate of 5 °C/minute. Elemental analyses (C, H and N) were recorded on a Thermo Finnigan EA 1112 with a Sartorius MC balance with a precision of ±0.2% at the Spectropole (Marseille, France). All the analyses were reproduced three times to confirm the organic content of the materials.
Solid-state 13C MAS experiments were performed at 7.05 T on a Bruker AVANCE 300 spectrometer using a 4 mm MAS Bruker probe. The Cross-Polarization MAS (CPMAS) pulse sequence was used with a contact time of 1 ms, spinal-64 1H decoupling and a spinning speed of 14 kHz. 13C chemical shifts were referenced to external adamantine (used as a secondary reference), the high frequency peak being set to 38.5 ppm.
For electrochemical characterization, electrodes were prepared using the plastic technology initially developed by Bellcore for Li battery electrode formation and implemented for sensor developments [37]. Typically, the sensitive material is manually ground with 17 wt.% of SP type carbon black (conducting additive), 25 wt.% of PVDF-HFP copolymer and 48 wt.% of DiButylPhtalate (DBP) plasticizer. Acetone is then added to the mixture so as to obtain a paste that can be cast over a glass plate. After drying, a plastic composite film is obtained, and can be cut to the required size according to the measurement to be performed. The film is then laminated on a platinum grid under a 20 psi pressure at 135 °C. Prior to being used, the DBP plasticizer is extracted using diethyl ether (two washings, each of 15 min). Open circuit voltage and potentiostatic measurements were carried out using a three-electrode cell configuration with a saturated calomel electrode (SCE) and Pt wires as reference and counter electrodes, respectively. For the potentiometric measurements, the three electrodes were immersed in aqueous FeCl3 solutions with concentrations ranging from 10–1 to 10–6 M after a 2-hour ageing period in a 10–2 M solution.
3 Results and discussion
As described above, the first step was to obtain the ionophore designed to interact with dissolved iron (III). For that, we used a bisICL670 substituted calix[4]arene in partial cone conformation to chelate the iron (III). The synthesis process was extensively described in a previous paper [30], and we will here only summarize the procedure (Fig. 2). The calix[4]arene was used as a platform with the incorporation of, firstly, two ICL670 molecules on the same side (for the formation of an hexadentate complex) and, secondly, an alkylamine function on the other side for the grafting on the silica particles. Starting with a calix[4]arene syn-1,3-diether protected with phtalimides, we introduced a single boc-protected alkylamine with a 30% yield leading to a 1,3-alternate conformation. Note that this conformation is “ideal” keeping in mind the grafting of the ionophore. After removing the phtalimides groups, peptidic couplings with ICL670 were realized and followed by the Boc group cleavage. The formed supramolecular system enables the complexation of iron (III) using the two tridentate ICL670 in a preformed conformation (L. Dupont, Private Communication) (Fig. 3).

Synthesis route of the modified ICL670-based calix[4]arene designed for iron (III) complexation; i: bromide, Cs2CO3, DMF; ii: aqueous hydrazine 35%, EtOH; iii: ICL670, HOBt, EDCI, CH2Cl2; iv: TFA, CH2Cl2.

Representation of iron (III) coordination with the bisICL modified calix[4]arene.
The second step for the building of hybrid materials sensitive to iron (III), consisted in the preparation of the inorganic matrix. For that, we have chosen to prepare the silica nanoparticles using the well-known Stöber's process [32]. Using a 70 °C temperature for the inorganic polymerization process, we obtained well-defined monodispersed silica nanoparticles (Fig. 4a). The average diameter, determined from image analysis, is 50 nm and consistent with previous studies of hydrolysis-condensation processes performed at 70 °C [38]. The following step was to link/couple the modified calix[4]arene with these nano-objects. The particle was organically modified with chloropropyl linkers (Fig. 5), by a reaction of a silylated precursor with the surface hydroxyl groups of silica. As expected, no modification of the particle size or morphology was observed (Fig. 4b). The final step consisted in a SN coupling of the propylamino modified calix[4]arene, with the chloropropyl-modified silica. The functionalization of the silica particles was first examined by FTIR analyses in DRIFT mode which gives enhanced chemical information from the surface as compared to classical transmission FTIR. As generally observed for a grafting process on preformed silica particles, we noticed the complete disappearance of the free silanol vibration (Fig. 6b) initially located at 3750 cm–1 (Fig. 6a). This indicates that these free silanols have totally reacted with the organosilane to form siloxane bridges. Then the calixarene derivatives were coupled with this surface organosilane, inducing quite small changes in the FTIR spectra (Fig. 7b). We similarly measured the spectrum of the native silica and the modified-calixarene prior to the grafting. From these spectra, we have a first clue of the grafting of the calixarene onto the silica spheres as underlined by the vibration bands between 1650 cm–1 and 1400 cm–1 corresponding to aromatic vibration bands of the calix[4]arene platform and ICL670 fragment.
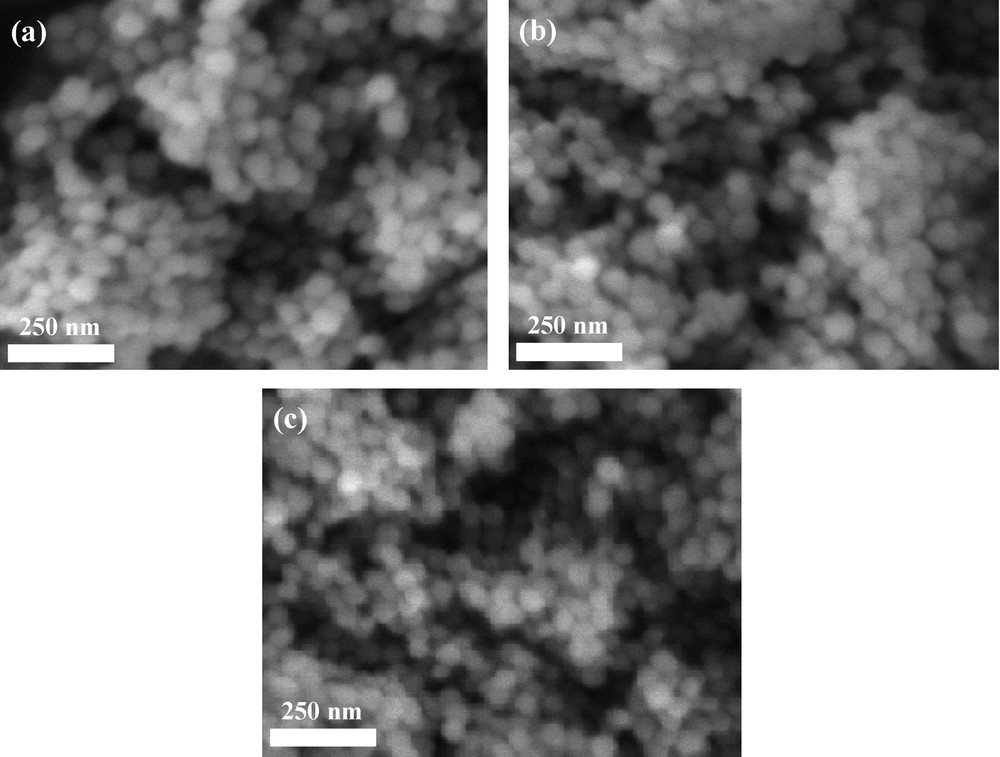
Scanning Electron Microscope (SEM) pictures of native silica particles (a), chloropropyl-modified silica particles (b), bisICL670 calix[4]arene-modified silica particles (c).

Synthesis scheme of the formation of the calix[4]arene-modified silica particles.
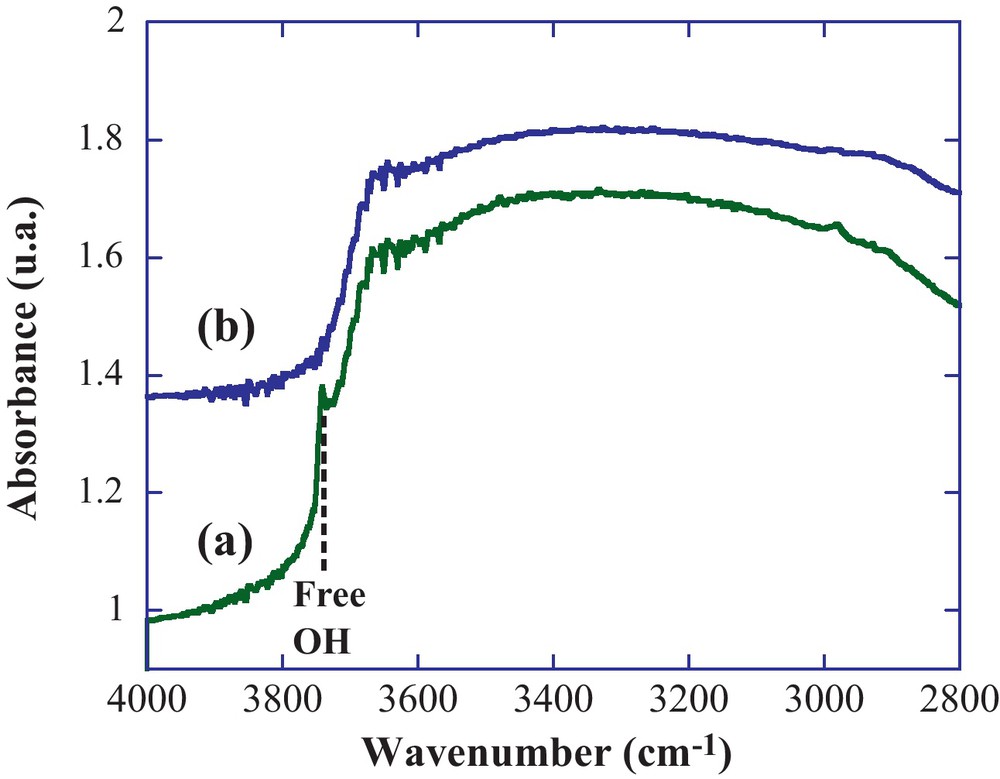
DRIFT spectra of native silica particles (a), bisICL670 calix[4]arene-modified silica particles (b).
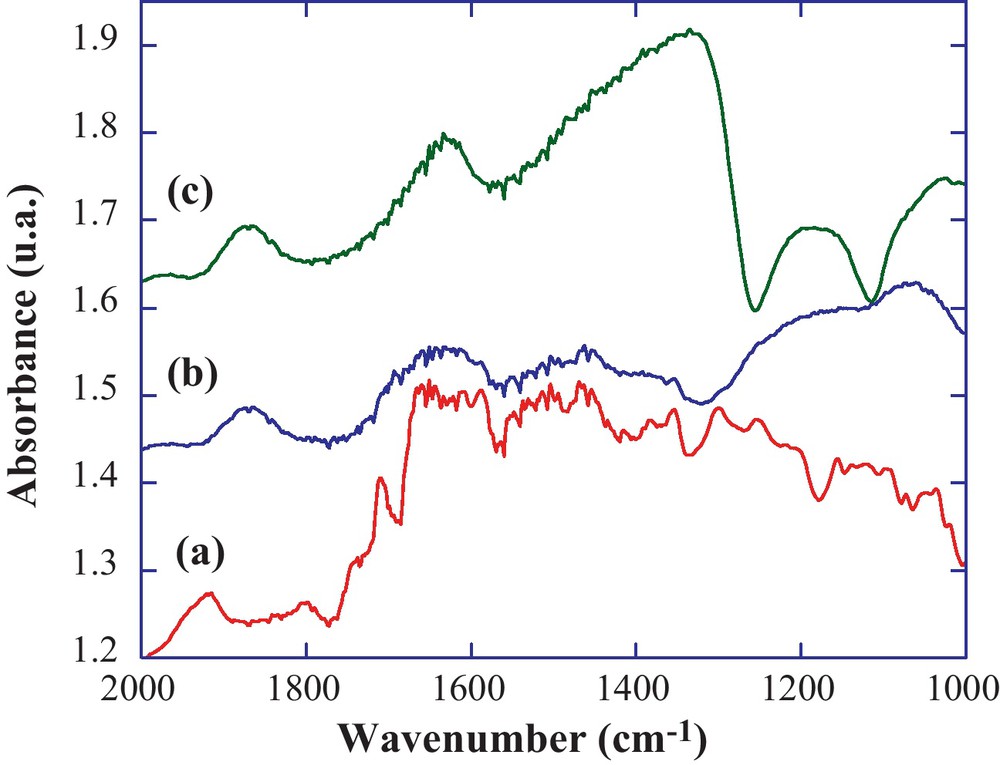
Comparison of the DRIFT spectra for bisICL670 calix[4]arene (a), bisICL670 calix[4]arene-modified silica particles (b), native silica particles (c).
A further proof of the successful functionalization process of the silica nanoparticles is brought by 13C solid-state NMR experiments. Fig. 8 gathers the spectra of the chloropropyl modified silica (a), the calix-functionalized particle (c) and for comparison the spectrum of the pure organochelator (b) (that is the bisICL670 propylamine calix[4]arene, represented on Fig. 3). These spectra were recorded at 14 kHz using a CPMAS sequence. Signals related to the chloropropyl fragment of the chloropropyl functionalized silica (Fig. 8a) are clearly observed at 11 ppm (CH2-Si), 28 ppm (central CH2) and 44 ppm (CH2-Cl) respectively, in agreement with previous works [39]. Additional peaks at 17 and 60 ppm arise from residual ethoxy groups resulting from incomplete hydrolysis of the sol-gel precursors (either TEOS, or the chloropropyl silane). After the coupling reaction, the obtained calix-Si-Nps material spectrum (Fig. 8c) shows the main signals of pure calixarene bisICL (Fig. 8b). At high chemical shift values, three characteristic broad peaks clearly observed at 170 to 152 ppm, 146 to 138 ppm and 136 to 113 ppm can be assigned to the carbonyl/phenolic quaternary carbon, triazole fragment resonance and the different ICL670 aromatic carbons, respectively. Furthermore, we can also distinguish a broad peak in the 65 to 82 ppm range corresponding to the CH2-O carbon of the alkyl oxide chains linked to the calixarene unit [30]. Besides, within the alkyl carbon region, we can distinguish a new peak at 35 ppm attributed to the secondary amine resulting from the anchoring of the calixarene species on the chloropropyl group of the organosilane. The covalent anchoring was also corroborated with the decrease of the Cl-CH2 peak initially observed at 44 ppm. Thus these spectra clearly demonstrate the successful binding of the modified calix[4]arene on the silica matrix.

Solid-state 13C Cross-Polarization MAS (CPMAS) NMR spectra of the chloropropyl-modified silica (a), bisICL670 calix[4]arene (b), the new hybrid material bisICL670 calix[4]arene-modified silica (c); *: residual ethoxy fragment.
We estimated the amount of calix[4]arene grafted on the silica nanoparticles by thermo-gravimetric analysis (Fig. 9). For the calculation, we subtracted the weight loss observed for the chloropropyl-silica from that of the final material. The 7.74% extra-loss corresponds to the replacement of the chlorine atom by the bisICL amine-modified calixarene and leads to 56 μmol of calixarene per gram of silica. This is confirmed by the elemental analysis results (C, H, N) from which we also estimated the calixarene content using either C, H or N composition. Using the nitrogen content, which is not initially present in the chloropropyl modified silica (Table 1), we can thus unambiguously conclude that we have grafted about 58 μmol of calixarene per gram of material, that is to say a grafting yield of 52% on the chloropropyl liner (about half of the present chloropropyl).
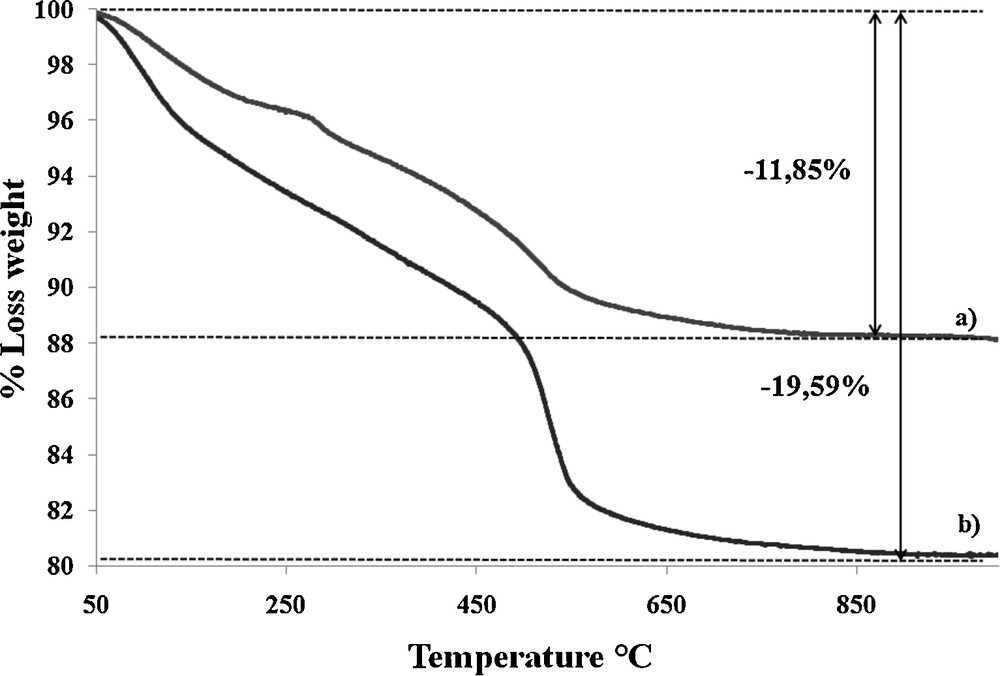
Comparison of the thermo-gravimetric curves obtained for a heating rate of 10 °C/min for the chloropropyl-modified silica (a), bisICL670 calix[4]arene-modified silica (b).
Elemental analysis obtained for the chloropropyl and calixarene-modified silica particles and subsequent estimation of the calixarene content.
%C | %H | %N | |
CP-Si-Nps | 4.84 | 1.23 | – |
Calix-Si-Nps | 10.32 | 1.71 | 0.74 |
Difference | 5.48 | 0.48 | 0.74 |
Calixarene content (μmol/g) | 55 | 64 | 58 |
Finally, we determined the ability of this original hybrid material to interact and detect iron (III) in solution. This was realized using the plastic Bellcore technology for the formation of the electrodes [40,41]. First, the electrodes were conditioned in a 10–2 mol.L–1 solution for 2 h prior to the measurements. Such a conditioning step is common for ion sensing and leads to a stable and reproducible electrode state. The open circuit potentials were then measured in solution with concentrations ranging from 10–2 to 10–6 mol.L–1. As seen on Fig. 10, the potential stabilized with a response time function of the concentration, namely the lower the concentration, the higher the response time. The reversibility of the equilibrium taking place during the measurement is good as the measure of the potential in a 10–2 mol.L–1 Fe (III) solution after the test gives back the pristine value. The open circuit voltage evolves linearly along the all tested concentration range showing that the prepared material can be used for quantitative determination of dissolved iron (III) with a sensitivity of 53 mV/decade of concentration (Fig. 11). The next step will be to evaluate the properties of this system in more complex media to evaluate, for instance, the selectivity of the electrode response and to optimise the analytical properties.
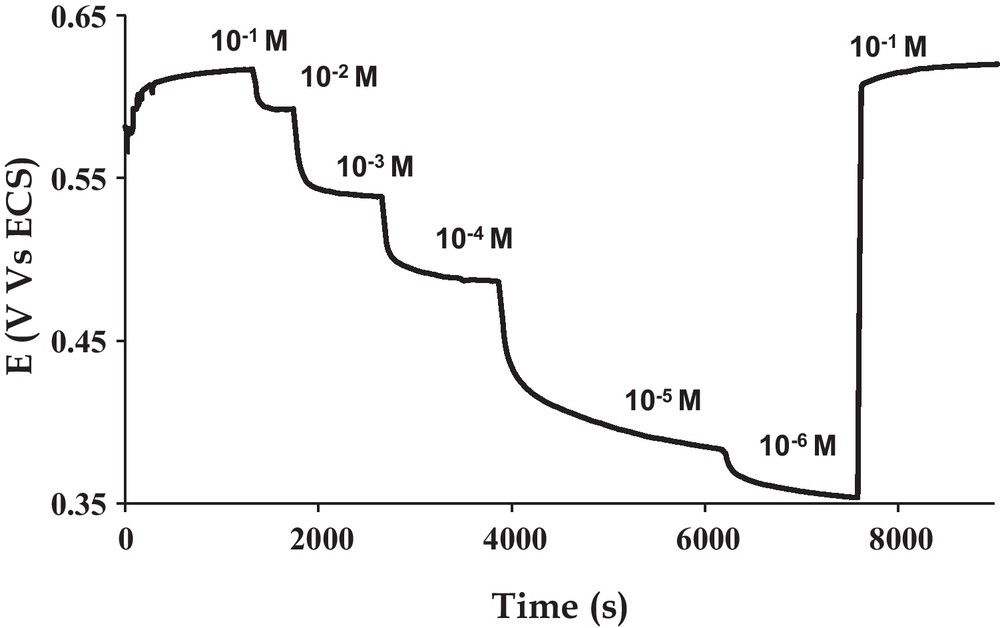
Response at 30 °C of bisICL670 calix[4]arene-modified silica-based plastic composite electrode as a function of iron (III) concentration.

Equilibrium potential of bisICL670 calix[4]arene-modified silica-based plastic composite electrode as a function of the logarithm of iron (III) ion concentration.
4 Conclusions
We presented, in this work, the preparation and characterization of a new hybrid material with the aim of forming new analytical tools for the potentiometric detection of iron (III) in solution. This material is made of silica nanoparticles obtained by the Stöber's process onto which an original iron's chelator was grafted. The chelator consists in a calixarene unit in a partial cone conformation modified with two iron (III) ligands (ICL670, Deferasirox). DRIFT and 13C NMR experiments unambiguously confirm the grafting of the calixarene. Finally, we studied the sensor ability of the hybrid material toward the iron III ion in aqueous solution. We observed a good linear potentiometric response of the material that can be attributed to the iron (III) complexation equilibrium taking place at the electrode surface. These promising results pave the way to a new kind of hybrid material which can allow the formation of analytical tool for ion sensing playing with both the matrix nature and the grafted macromolecules.
Acknowledgements
The authors thank Dr. Mohammed Benazza for enlightening discussions.