1 Introduction
The use of water as a solvent for organic reactions has been important since the pioneering studies of Diels–Alder reactions by Breslow [1]. There has been increasing recognition that organic reactions can proceed well in aqueous media and offer advantages over those occurring in organic solvents [2]. Water has interesting physiochemical properties, which include high thermal capacity, hydrophobic association, high polarity, and hydrogen-bonding ability [3–7]. Due to these properties, the use of water as a reaction medium for clean catalytic transformations would have profound effects on reaction rates and product selectivity. Quinoxaline derivatives have attracted much attention owing to their biological activities. They display a broad range of biological, medicinal, and pharmacological properties and are constituents of antiviral, antibacterial, anti-inflammatory, and antiprotozoal drugs and as kinase inhibitors [8–12]. Their utility as dyes, electroluminescent materials, organic semiconductors, cavitands, chemically controllable switches, DNA cleaving agents and many other applications are well documented [13–18]. In particular, quinoxaline drugs, such as clofazimine, echinomycin, leromycin and actinomycin are some of the leading ones on the market with diverse functionalization around the quinoxaline motif [19–24]. In view of the great importance of quinoxaline derivatives, in recent years, efforts have been made in developing new methodologies for the synthesis of these compounds [25]. The condensation of aryl 1,2-diamines with 1,2-dicarbonyl compounds represents an important existing procedure for the synthesis of 2,3-disubstituted quinoxalines.
Generally, this condensation has been carried out under reflux conditions in ethanol or acetic acid [26]. In recent years, several new efficient methods have been developed, including the use of β-cyclodextrin (β-CD) [27], ionic liquids [28], molecular iodine [21], heteropolyacid [29], cellulose sulfuric acid [30], Zn[(l)proline] [31], hypervalent iodine(III) sulfonate in PEG [32], polyaniline-sulfate salt [33], DABCO [34], CAN [35], fluorinated alcohols [36] and (NH4)6Mo7O24·4 H2O [37]. These methods show varying degree of successes as well as limitations, such as harsh reaction conditions, expensive and detrimental metal reagents, tedious work-up, low product yields, long reaction times, and co-occurrence of several side products. Therefore, a simple, efficient method for quinoxaline synthesis remains an attractive goal. Recently Zhang et al. have found that gallium(III) triflate can catalyze successfully the reactions of phenylene-1,2-diamines and 1,2-diketones under mild conditions [38]. This method was suitable for a wide range of substrates, and especially for functionalized phenylene-1,2-diamines. Yadav et al. have reported a one-pot synthesis of phenylene-1,2-diamine derivatives by cyclocondensation of arene-1,2-diamines with 1,2-dicarbonyls using a catalytic amount of bismuth(III) triflate [39]. The use of water as a solvent makes this procedure attractive and environmentally benign. However, realizing the fact that metal-free organocatalysis has drawn considerable interest from chemists, and that metal-free homogeneous catalysis is advantageous for designing suitable drugs devoid of any metal content, it would be desirable to develop this reaction using metal-free Lewis acid catalysts [40].
Catalysis with small organic molecules, where an inorganic element is not part of the active principle, has become a highly dynamic area in chemical research [41]. Such catalysts have several important advantages, since they are usually robust, highly reactive, eco-friendly, inexpensive, readily available, and non-toxic [42–44]. As an inexpensive and commercially available organocatalyst, pentafluorophenylammonium triflate (C6F5N+H3*TfO–; PFPAT) has received increasing attention as a water-tolerant Brønsted acid catalyst for organic synthesis demonstrating highly chemo-, regio- and stereoselective results [45–47]. Due to the current challenges for developing environmentally benign synthetic processes and in continuation of our interest in the application of new organocatalysts for various organic transformations [48–53], we report an efficient route for the synthesis of quinoxaline derivatives using pentafluorophenylammonium triflate (PFPAT) as a catalyst (Scheme 1).
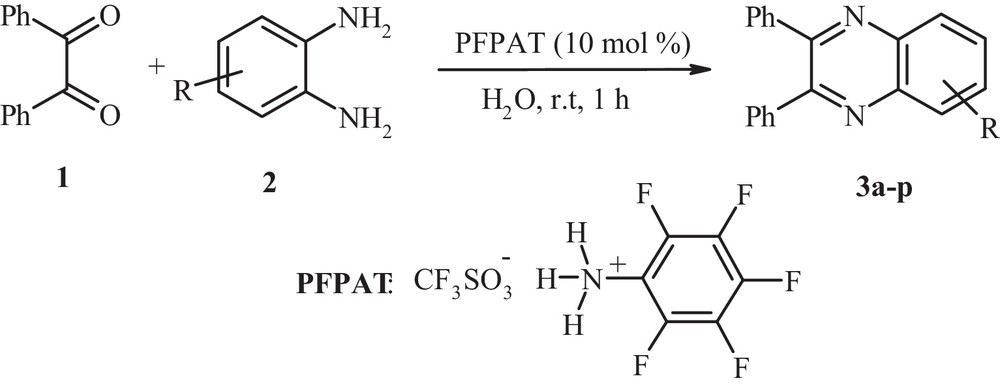
Synthesis of 2,3-disubstituted quinoxalines derivatives in water.
2 Results and discussion
In order to optimize the reaction conditions, we chose the condensation of the reaction of benzil (1 mmol) with o-phenylenediamine catalyzed by PFPAT under different conditions both in the absence and in the presence of PFPAT; the results are given in Table 1. It is noteworthy that in the absence of the catalyst, the reaction was rather sluggish in water and resulted in very low yields (20–35%) of quinoxalines, even after long reaction times (Table 1, entry 1). Then, the effects of temperature, the amount of catalyst, and the reaction time on the yield of the product were examined. Reaction at room temperature in water in the presence of 30 mg (10 mol%) PFPAT afforded the product 3 in 95% yield (Table 1, entry 3). Increasing either the amount of catalyst and/or prolonging the reaction time did not improve the yield (Table 1, entry 9), while reducing these factors led to a reduction in the product yield (Table 1, entry 2). Further studies confirmed that 10 mol% of PFPAT was optimum for this reaction and gave a product in 95% yield in just 1 h (Table 1, entry 3). The reaction was also examined in solvents such as toluene, THF, CH2Cl2, ethanol, and diethyl ether. When the reaction was carried out in the presence of PFPAT in water, the expected product (3a) was obtained in high yield (95%) and with better reaction times compared with other organic solvents (Table 1, entries 3–8). With non-polar diethyl ether and toluene, the desired adduct was not produced. Moreover, when the reaction was carried out in water, the solid product was separated at the end of the reaction.
Effects of the amount of catalyst PFPAT used and of the solvent on the formation of 3.
Entry | PFPAT amount (mol%) | Condition/solvent | Time (h)/yield |
1 | 0 | rt/H2O | 6/30 |
2 | 5 | rt/H2O | 5/80 |
3 | 10 | rt/H2O | 1/95 |
4 | 10 | rt/CH2Cl2 | 5/10 |
5 | 10 | rt/THF | 5/20 |
6 | 10 | rt/ethanol | 5/60 |
7 | 10 | rt/toluene | 5/10 |
8 | 10 | rt/diethyl ether | 10/10 |
9 | 20 | rt/H2O | 1/95 |
At the beginning of the reaction, the reagents itself were dissolved completely in the medium to form a homogeneous mixture (Fig. 1a), but near the completion of the reaction, the system became a suspension, and the product precipitated at the end of the reaction (Fig. 1b).
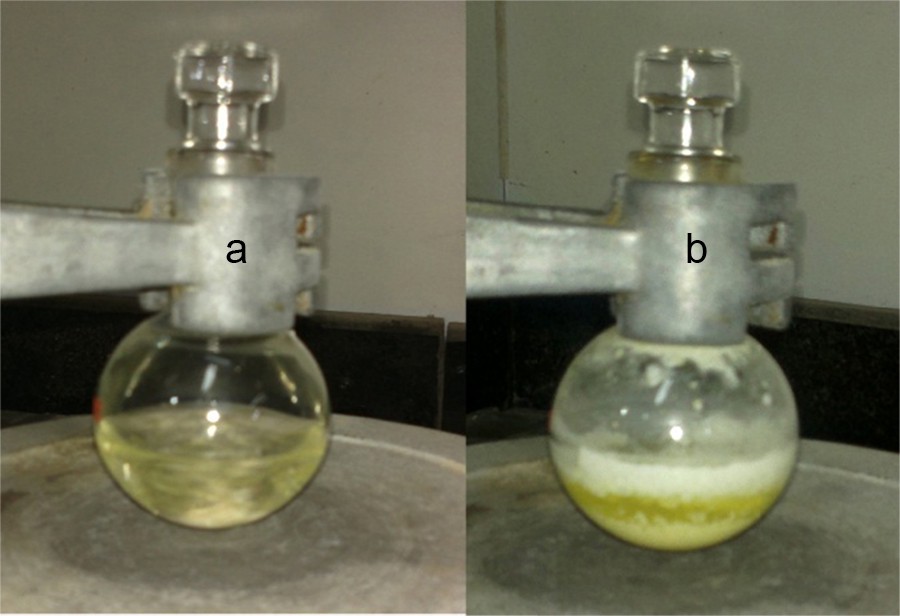
(Color online.) (a) Homogeneous mixture during the reaction, and (b) at the end of the reaction; the product has precipitated.
The products were obtained through simple filtering, and recrystallized from hot ethanol to afford pure products. The corresponding functionalized quinoxalines-scaffolds 3a, shown in Table 1 and confirmed by NMR measurements, were obtained in good yield (95%).
Further experiments revealed that a similar procedure is applicable for the preparation of a wide range of compounds analogous to adduct 3 (Table 2). In order to evaluate the efficiency of this methodology, various arene-1,2-diamines, such as mono- and disubstituted amines, reacted efficiently with 1,2-dicarbonyl compounds to give the corresponding 2,3-disubstituted quinoxalines (Table 2). The results displayed in Table 2 show that electron-donating groups at the phenyl ring of 1,2-diamine favored the formation of product (Table 2, entries 2 and 3) to give quantitative yields. In contrast, electron-withdrawing groups, such as chloro- and bromo- gave slightly lower yields (85–87%) (Table 1, entries 4 and 5). Interestingly, 2,3-diaminopyridine underwent smooth coupling with benzil to afford the corresponding pyrido[2,3-b]pyrazine 3f in 80% yield (Table 2, entry 6). Similarly, several 1,2-dicarbonyl compounds, such as furil, 1,2-di(4-chlorophenyl)ethanedione, phenylglyoxal, ninhydrin and acenaphthene-1,2-quinone also reacted rapidly with 1,2-diamines to produce a variety of quinoxaline derivatives (Table 2, entries 8–19). In all cases, the reactions proceeded rapidly at room temperature with high efficiency. The products were characterized by 1H and 13C NMR, IR spectroscopic data and also by comparison with authentic samples. This method not only affords the products in excellent yields, but also avoids the problems associated with catalyst cost, handling, safety, and pollution. One of the major advantages of this protocol is the isolation and purification of the products, which have been achieved by simple filtration and crystallization of the crude products.
Quinoxaline derivatives from the reaction of 1,2-diamines and 1,2-diketones catalyzed by PFPAT.
Entry | Dicarbonyls | Diamines | Product | Yield (%) | Entry | Dicarbonyls | Diamines | Product | Yield (%) |
1 | 3a | 95 | 11 | 3k | 90 | ||||
2 | 3b | 95 | 12 | 3l | 95 | ||||
3 | 3c | 92 | 13 | 3m | 85 | ||||
4 | 3d | 85 | 14 | 3n | 80 | ||||
5 | 3e | 87 | 15 | 3o | 90 | ||||
6 | 3f | 80 | 16 | 3p | 85 | ||||
7 | 3g | 80 | 17 | 3q | 90 | ||||
8 | 3h | 95 | 18 | 3r | 85 | ||||
9 | 3i | 95 | 19 | 3s | 90 | ||||
10 | 3j | 90 |
The efficiency of various catalysts in the synthesis of quinoxalines derivatives has been compared in Table 3. The best yield and the shorter reaction time are attributed to the high efficiency of the PFPAT organocatalyst.
Comparison the results of the synthesis of 2,3-diphenylquinoxaline using different catalysts.
Entry | Catalyst | Time | Yield (%) | Temperature (°C) | Reference |
1 | montmorillonite K10 | 2.5 h | 100 | rt | [54] |
2 | I2/DMSO | 35 min | 95 | rt | [20] |
3 | Zn2+-K10-clay (clayzic) | 24 h | 98 | rt | [55] |
4 | cellulose sulfuric acid | 2.30 h | 80 | rt | [30] |
5 | Keggin type heteropolyacids | 1 h | 92 | rt | [29] |
6 | MeOH/AcOH | 5 min | 99 | 160 | [56] |
12 | PFPAT | 1 h | 95 | rt | This work |
A plausible reaction mechanism and the role of PFPAT are depicted in Scheme 2. In this process, PFPAT acts as Brønsted acid and plays a significant role in increasing the electrophilic character of the electrophiles (Scheme 2).
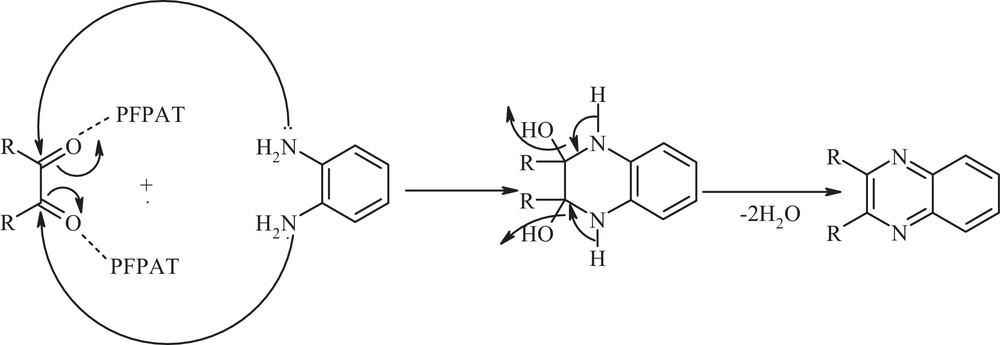
Proposed mechanism for the synthesis of quinoxaline.
The recovered PFPAT from the aqueous layer was reused in three subsequent runs with a gradual decrease in activity. For example, the treatment of benzil (1) with o-phenylenediamine (2) in the presence of 10 mol% PFPAT in water (4 mL) for 60 min gave the desired product 3a in 95%, 90%, and 88% yields over three cycles.
We believe that the procedure is simple, convenient and does not require any aqueous work-up, thereby avoiding the generation of waste, and may contribute to the area of green chemistry.
3 Conclusions
In conclusion, a simple and highly efficient method for the synthesis of quinoxaline derivatives through the reaction of aryl 1,2-diamines with 1,2-dicarbonyl compounds at room temperature catalyzed by pentafluorophenylammonium triflate in water at room temperature has been proposed in this work. In contrast to the existing methods using potentially hazardous catalysts/additives, the present method offers the following competitive advantages:
- • PFPAT is easy to prepare from commercially available pentafluoroaniline and triflic acid;
- • the reaction proceeds with short reaction time;
- • the product is easy to isolate/purify;
- • there are no side reactions;
- • handling and processing are cheap and simple;
- • quinoxaline derivatives are produced through an environmentally benign process.
4 Experimental
4.1 Typical experimental procedure
A mixture of 1,2-dicarbonyl compounds (1 mmol), aryl 1,2-diamines (1 mmol) dissolved in 4 mL water, and PFPAT (10 mol%) was stirred for the appropriate reaction time. The reaction was monitored by TLC. After completion of the reaction (monitored by TLC), the resultant was cooled with ice-salt bath, filtered and washed with ethanol and purified by recrystallization from hot ethanol to afford pure products 3a–p, and the filtrate containing PFPAT could be directly used by adding the reactants. After three recycles, the catalytic activity of PFPAT remained unchanged. The products were characterized by comparison of their physical and spectral data with those of authentic samples. Spectroscopic data for the selected examples are shown below. Spectroscopic data for selected examples as follows.
4.1.1 2,3-Diphenylquinoxaline
White solid; mp 126–127 °C; IR (KBr): 3051, 1630, 1528, 1348, 772 cm−1; 1H NMR (CDCl3, 400 MHz): δ = 7.33–7.41 (m, 6H), 7.53–7.57 (m, 4H), 7.76–7.83 (m, 2H), 8.20–8.23(m, 2H); 13C NMR (100 MHz, CDCl3): δ = 128.2, 128.9, 129.2, 129.8, 129.9, 139.1, 141.2, 153.4 (Table 1, entry 1).
4.1.2 6-Methyl-2,3-diphenyl-quinoxaline
White solid; mp 118–120 °C; IR (KBr): 3052, 1640, 1532, 1344, 775 cm−1; 1H NMR (CDCl3, 400 MHz): δ = 2.6 (s, 3H); 7.32 (m, 6H); 7.52 (m, 4H); 7.57 (d, J = 8.75 Hz, 1H); 7.96 (s, 1H); 8.05 (d, J = 8.5 Hz, 1H); 13C NMR (100 MHz, CDCl3): δ = 21.9, 127.1, 128.2, 128.6, 128.7,129.8, 132.3, 139.2, 139.7, 140.4, 141.3, 152.5, 153.3 (Table 1, entry 2).
4.1.3 2,3-Diphenylpyrido[2,3-b]pyrazine
Yellow crystals; mp 135–137 °C; IR (KBr): 3055, 1640, 1530, 1340 cm−1; 1H NMR (400 MHz, CDCl3): δ = 7.34–7.45 (m, 5H), 7.59–7.66 (m, 4H), 7.73–7.76 (m, 2H), 8.54–8.56 (m, 1H), 9.20 (d, J = 4.8 Hz, 1H); 13C NMR (100 MHz, CDCl3): δ = 125.6, 128.5, 128.8, 129.7, 129.8, 130.2, 130.6, 136.6,138.5, 138.9, 150.2, 154.4, 155.1, 156.7 (Table 1, entry 6).
4.1.4 2-Furan-3-yl-3-furan-2-yl-quinoxaline
Light yellow solid; mp 134 − 136 °C; IR (KBr): 3427, 2983, 1605, 1567, 1442, 879, 743 cm−1; 1H NMR (400 MHz, CDCl3): δ = 6.68–6.53 (m, 4H), 7.68–7.63 (m, 2H), 7.80–7.73 (dd, J = 3.1 Hz, J = 6.8 Hz, 2H), 8.18–8.11 (dd, J = 3.5 Hz, J = 6.3 Hz, 2H); 13C NMR (125 MHz, CDCl3): δ = 112.1, 113.5, 128.1, 133.6, 142.2, 143.4, 145.1, 150.1 (Table 1, entry 8).
4.1.5 2-Furan-3-yl-3-furan-2-yl-6-methyl-quinoxaline
Light yellow solid; mp 116 − 118 °C; IR (KBr): 3422, 2980, 1600, 1567, 1444 cm−1; 1H NMR (400 MHz, CDCl3): δ = 2.57 (s, 3H), 6.68–6.65 (m, 4H), 7.59–7.54 (dd, J = 1.8 Hz, J = 8.6 Hz, 1H), 7.64–7.60 (m, 2H), 7.93–7.88 (s, 1H), 8.04–7.99 (d, J = 8.6 Hz, 1H). 13C NMR (100 MHz, CDCl3): δ = 21.6, 21.9, 111.9, 112.6, 112.8, 117.2, 118.9, 124.6, 127.9, 128.6, 132.8, 141.2, 150.9 (Table 1, entry 9).
4.1.6 Acenaphtho[1,2-b]quinoxaline
White solid; mp 242–245 °C. IR (KBr): 3443, 3047, 2922, 2361, 1614, 1481 cm−1. 1H NMR (400 MHz, CDCl3): δ = 7.70–7.75 (m, 2 H), 7.98 (t, J = 7.7 Hz, 2 H), 8.15 (d, J = 7.7 Hz, 2 H), 8.18–8.22 (m, 2 H), 8.42 (d, J = 7.7 Hz, 2 H); 13C NMR (100 MHz, CDCl3): δ = 125.7, 126.8, 127.2, 128.2, 128.9, 129.6, 130.1, 133.2, 142.5, 145.3 (Table 1, entry 11).
Acknowledgment
This research is supported by the Islamic Azad University, Ayatollah Amoli Branch.