1 Introduction
Polyacrylamide (PAAm) stands as a versatile reactive polymer, largely due to its amide functionality. The amino group of the amide functionality facilitates the cross-linking of PAAm, and, interestingly, the resulting network has been efficient in the decontamination of soil and surfaces polluted by cesium and strontium following a nuclear accident [1]. Aminophenyl glycosides have been chemically attached to polyacrylamide to serve as affinity columns in cell filtration [2]. In its grafted form, PAAm was transformed into polyvinylamines as ion exchangers and flocculants by Hofmann degradation and Mannich reaction [3–5]. Sulfomethylated high-molecular-weight PAAm was obtained by reaction with formaldehyde and bisulfate [6]. The N-alkylation of the amide groups of polyacrylamide with n-alkyl bromide was conducted in DMSO and in the presence of potassium tert-butoxide [7]. PAAm-bound Schiff bases were employed as ligands for cobalt complexes, which are designed for the selective oxidation of olefins and alkyl halides [8]. PAAm hydrogels have been generated by hydrophobic modification via alkyl chains [9]. Polyacrylamide can be homolytically functionalized on its polyethylenic chain when a γ-irradiation source is applied. The latter technique was successful for grafting poly(4-vinylpyridine) onto PAAm [10]. Poly(N-2-aminoethylacrylamide) from the transamidation of PAAm with ethylenediamine was used as a chelating material for liquid chromatography [11]. However, polymers bearing polyhydroxylated benzene moieties, namely dihydroxybenzenes and their corresponding benzoquinones, display distinct properties and have been utilized in various applications [12–14]. Producing poly(vinyl-polyhydroxybenzene)s, such as poly(vinylhydroquinone), is unfortunately not a straightforward task, as the phenol functionality is sensitive to the polymerization conditions; moreover, it is typical to protect this functionality in the vinyl monomer prior to polymerization and to deprotect it afterwards.
Thus, incorporating polyhydroxylated benzene onto a premade polymeric matrix has been considered as a good alternative. Yoshida et al. [15] prepared a polymeric material from a ligninocatechol by means of a laccase-catalyzed polymerization, an environmentally friendly procedure. McGrath and co-workers [16] showed the potential applications of a partially disulfonated hydroquinone (HQ)-based poly(arylene ether sulfone) random copolymer as a proton exchange membrane. Gurnule et al. [17] evaluated 2,4-dihydroxybenzophenone-oxamide-formaldehyde terpolymers as ion-exchanging resins for Cu(II), Hg(II), Cd(II), Co(II), Zn(II), Ni(II), Pb(II), and Fe(III).
To continue our ongoing work on the functionalization of polyacrylics [18–21], we report herein the results of the chemical modification of polyacrylamide (PAAm) with a hydroquinone (HQ) entity by applying the conditions of Minisci [22] and the metallic adsorption properties of PAAm and the modified polymer.
2 Experimental
2.1 Methods and materials
Polyacrylamide was prepared according to a previously reported procedure [21]. Ammonium persulfate ((NH4)2S2O8) was recrystallized from water. 1,4-Benzoquinone (BQ), silver nitrate (AgNO3), copper nitrate Cu(NO3)2.3H2O, lead nitrate Pb(NO3)2, and cadmium nitrate Cd(NO3)2.4H2O were used as purchased. Chemicals were purchased from one of the following suppliers: Aldrich, Fluka AG, Prolabo, and Merck.
UV–Vis spectra were recorded using a JASCO UV–Vis spectrophotometer; bi-distilled water was employed as the solvent. Infrared spectra were taken with a JASCO 4200 FT–IR instrument. Polymer samples used for infrared analysis were in the form of cast films. 13C NMR spectra were recorded using a Bruker 400 MHz spectrometer at the Laboratoire Méthodologie RMN (FR CNRS 2843, UMR UHP-CNRS CRM2 7036), Université Henri-Poincaré (UHP) (France) with D2O as the solvent. XRD analysis of thin films were recorded using a Bruker D8 X-ray diffractometer (radiation Cu Kα, λ = 0.154 nm, 40 kV, 100 mA), at a 2θ grazing angle from 5° to 90° at a scan rate of 0.02°/s. SEM images of the polymers were taken using a JOEL JSM-6700F scanning electron microscope operating at an accelerating voltage of 3 kV. DSC thermograms were recorded on a Netzsch DSC 204 F1 fitted with an electronic module for nitrogen liquid cooling. Temperature and enthalpy calibrations were set with indium, and an empty aluminum pan was taken as a reference. Samples of weights in the 5–10-mg range were heated up to 120 °C at a heating rate of 20 °C/min under nitrogen flow, followed by quenching to 25 °C using liquid nitrogen. The DSC curves were recorded during the second heating cycle at a heating rate of 5 °C/min up to 250 °C, and the values of Tg were then determined according to the tangent method. TGA curves were recorded on a TGA Q500 thermogravimetric analyzer coupled with FT–IR. The polymer weight samples were in the 2.5–25-mg range, and the thermogravimetric analyzer was scanning a temperature domain from 20 to 1000 °C at a heating rate of 10 °C/min under a nitrogen flow rate of 25 mL/min.
The average molecular weights were measured by means of size (steric) exclusion chromatography using an Ultimate 3000 DIONEX system equipped with four 30-cm-high in-a-row columns and with a Shodex OH-pack type (803HQ, 804HQ, 806HQ, 807HQ), a differential refractometer OPTILABrEX (Wyatt Tech), and a multi-angle light scattering detector (MALS) DAWN HELEOSII (Wyatt Tech). The eluent was a 0.1 M NaNO3 aqueous solution, with a flow rate of 0.5 mL/min. The injected volume was 0.1 mL of a 2 to 3 g/mL polymer solution.
A Varian 720-ES plasma torch spectrometer was employed to assess the extent of metallic adsorption by resins, providing an argon flow rate of 15 L/min and a generator power of 1.2 kW.
2.2 Typical protocol for the modification reaction of polyacrylamide
In a 500-mL round-bottom flask equipped with a condenser, 0.5 g (7.04 × 10−3 mol) of PAAm was dissolved into 50 mL of distilled water, followed by addition of 50 mL of an ethanolic solution containing 3.85 g (3.56 × 10−2 mol) of p-benzoquinone (BQ). To this homogeneous mixture, 0.039 g (2.3 × 10−4 mol) of silver nitrate was added, followed by addition of 150 mL of distilled water. The system was heated to 75–80 °C under magnetic stirring. Once this temperature was reached, a saturated solution of ammonium persulfate (1.88 g, 8.24 × 10−3 mol) was added dropwise. The reaction was stopped, and the contents were cooled to room temperature. The modified PAAm was isolated in the following sequential steps:
- • evaporation of ethanol followed by addition of 50 mL of distilled water;
- • allowing the mixture to stand for one day to assure the precipitation of unreacted BQ (the precipitate was eliminated by filtration);
- • obtaining the modified PAAm by casting from the solution within 4 days. The cast film was then dried in vacuo at 45 °C for 4 days.
The degree of substitution, S (%), was provided by Eq. (1):
(1) |
(2) |
- • n2 is the number of moles of HQ (in the form of vinyl hydroquinone) present in the modified polyacrylamide, estimated from the UV calibration curve of hydroquinone;
- • n1 is the number of moles of acrylamide (AAm) present in the modified PAAm, provided by Eq. (2);
- • m is the sample weight of the HQ–PAAm used in the UV measurements;
- • M1 and M2 are the molecular weights of AAm and vinyl hydroquinone, respectively.
2.3 Metallic ion adsorption
2.3.1 Preparation of the resins
Into a 100-mL round-bottom flask fitted with a reflux condenser, 5 g (7.04 × 10−2 mol) of polymer were charged and dissolved into 100 mL of water. An amount of 0.39 g (2.3 × 10−3 mol) of AgNO3 was then added, and the mixture was heated under stirring at a temperature of 70–75 °C. A 100-mL aqueous solution of 1.88 g (6.96 × 10−3 mol) of K2S2O8 was then added dropwise to the mixture. The latter was left under stirring in this temperature range for almost one hour, at which time a precipitate was observed. The precipitate was isolated and washed abundantly with water and was dried to constant weight in vacuo for 4 days at 45 °C.
2.3.2 Typical procedure for metallic adsorption/desorption
In a thermostated cell, 0.1 g of cross-linked resin was added to 50 mL of a 50 mg/L Cu(NO3)2 aqueous solution, which had been previously adjusted to the desired pH (with 0.1 N HCl and 0.1 N NaOH). The system was stirred with a magnetic bar at 25 °C. Sampling of the supernatant liquid was performed at specified time intervals. The adsorption capacity Q (in mg of copper/g of resin) was computed from Eq. (3):
(3) |
Desorption of metal ions from the dried loaded resin (0.2 g) was conducted using 0.1 N HNO3 (50 mL) at 25 °C for 2 h. The regenerated resin was subjected to two additional adsorption–desorption cycles. The desorbed metallic ions were estimated by ICP–ES (inductively coupled plasma-emission spectroscopy). The desorption extent R (%) was calculated from Eq. (4):
(4) |
3 Results and discussion
3.1 Functionalization of PAAm
Polyacrylamide having the characteristics shown in Table 1 was prepared as previously reported [21]. PAAm underwent chemical modification with 1,4-benzoquinone under the oxidative decarboxylation conditions of Minisci [22], as indicated in Eq. (5). The mechanism proposed for such chemical modification is illustrated in Scheme 1. In this mechanism, it is proposed that the amide groups of PAAm underwent hydrolysis under the conditions shown, to give rise to carboxylic groups. Notably, enzymatic hydrolysis of acetamide, benzamide, and butyramide yielded their corresponding carboxylic compounds [23]. The (NH4)2S2O8/AgNO3 system then induced the formation of a polymeric radical PAAm species by oxidative decarboxylation. As an electron-rich site behaving as a nucleophilic alkyl, the PAAm radical species readily reacted with benzoquinone compounds, which are considered to be electron-deficient species. Minisci advanced the latter mechanistic explanation for the homolytic alkylation of heteroaromatic substances [24,25]. Nucleophilic alkyl radicals were reported to preferentially attack the carbon atom of the benzoquinone compound [24].
Molecular weights and thermal properties of PAAm and HQ–PAAm.
Polymer | (g/mol) | (g/mol) | Tg (°C) | Tm (°C) | |
PAAm | 8.30 | 3.03 | 2.73 | 161.19 | – |
HQ–PAAm* | 5.06 | 2.14 | 2.37 | 78.82 | 240 |
* HQ–PAAm from [BQ]/[AAm] = 5; the degree of substitution was 58.49%.
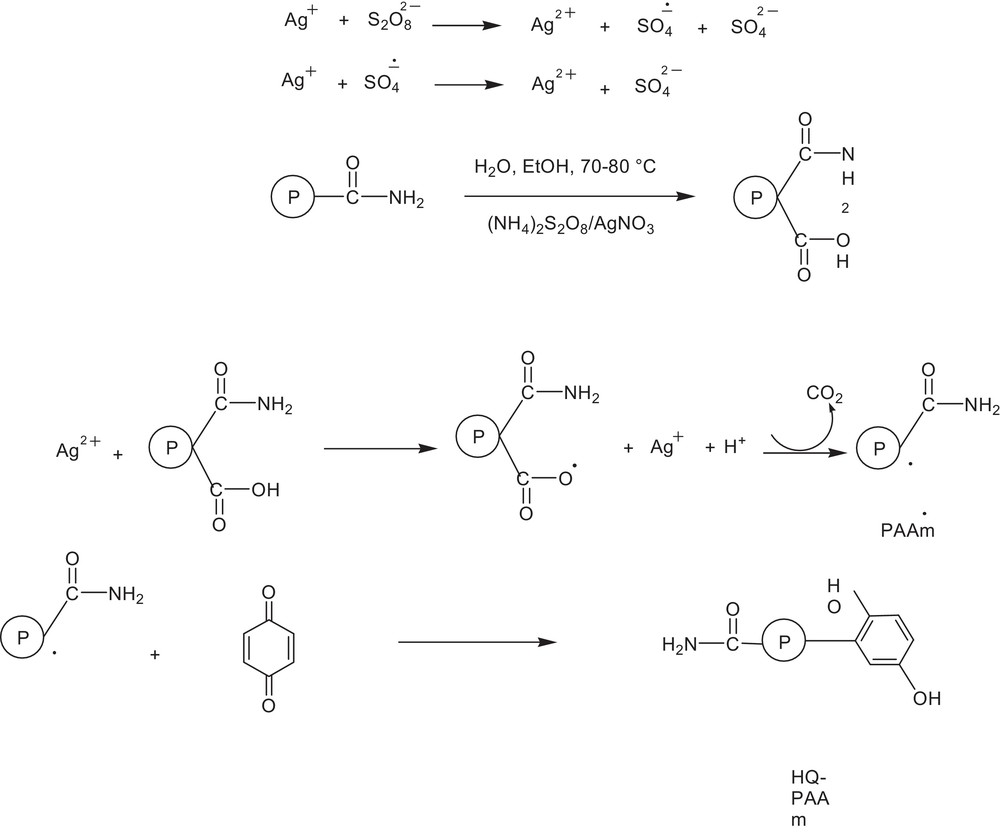
Proposed mechanism for PAAm modification.
The success of chemical modifications of PAAm with HQ was confirmed by UV–visible, FT–IR and 13C NMR spectroscopies (Figs. 1–3). The UV–visible spectrum of modified PAAm (Fig. 1) reveals a new absorption band centered at λmax = 280 nm, which is a featured band of the hydroquinone unit. Additionally, infrared analysis (Fig. 2) revealed new absorption bands for HQ–PAAm compared to those for virgin PAAm. The carbonyl absorption band of the amide is substantially decreased, suggesting a higher degree of substitution and deamidation as explained mechanistically. The characteristic bands of the HQ moiety appeared clearly in the FT–IR spectrum (Fig. 2b):
- • two sharper bands at 860–870 and 1200–1250 cm−1, attributable to the angular deformation of CC and to the stretching of CO, respectively;
- • a band at 1400–1425 cm−1, assigned to the planar CH band of the benzene ring;
- • a wide band at approximately 3400 cm−1, ascribed to the OH band.
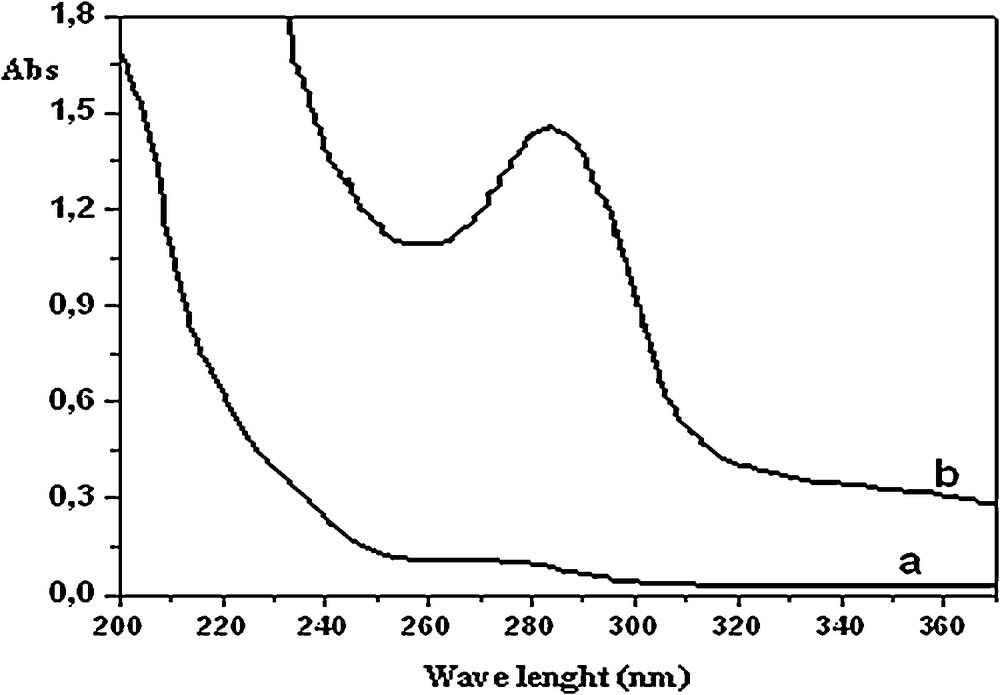
UV–visible spectra: a: PAAm; b: HQ–PAAm ([BQ]/[AAm] = 5). Solvent: bi-distilled water.

(Color online). FT–IR spectra: a: PAAm; b: HQ–PAAm ([BQ]/[AAm] = 5).
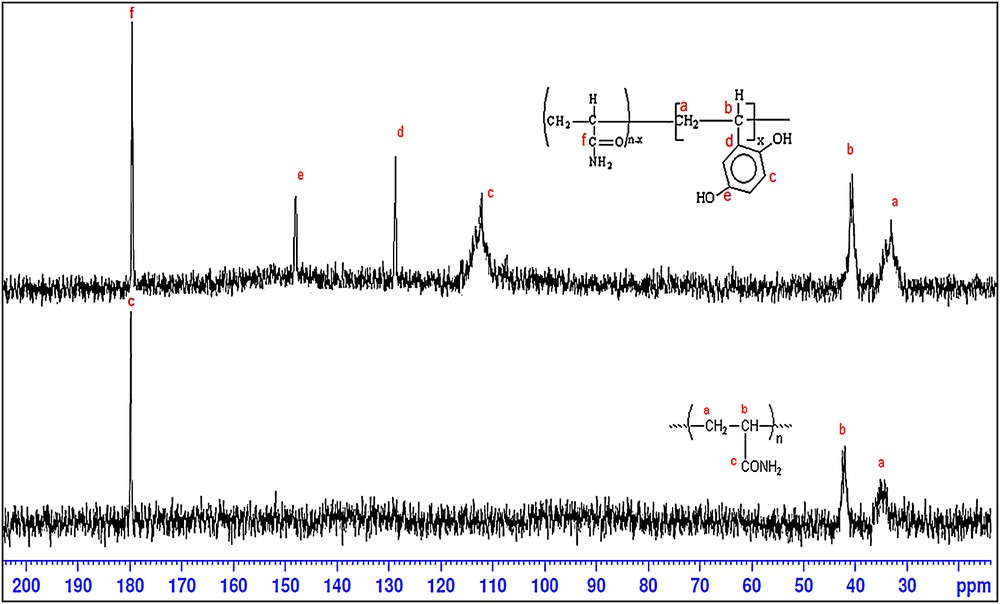
(Color online). 13C NMR spectra of PAAm and HQ–PAAm. D2O as solvent.
Analysis by 13C NMR spectroscopy further supported the success of the polyacrylamide chemical reaction (Fig. 3). Indeed, besides the chemical shifts of different carbons of PAAm appearing near 36, 42, and 180 ppm, those of the HQ units appear near 115 (broad), 130, and 151 ppm.
An unexpected result of such modification was the decrease in molecular weight of the polymer after modification; indeed the molecular masses and of HQx–PAAm (x = 0.58, the degree of substitution) were measured as 5.06 × 106 and 2.14 × 106 g/mol (Table 1), respectively. That is, the mass of the modified polymer decreased by approximately 60% from the initial molecular weight of PAAm, suggesting severe degradation over the course of the modification reaction. This fact had been previously observed when polyacrylics were modified with polyhydroxylated benzenes under identical conditions [18–21]. This reduction in molecular weight remains largely unexplained, but radical chain scission may be part of the degradation-inducing phenomenon. However, the glass transition temperature for the modified PAAm (Tg = 78.82 °C) was drastically lower than that for the pristine PAAm (161.19 °C), as it is evident from Fig. 4 (and Table 1), thus suggesting a degradation of the polymeric matrix upon grafting HQ groups. An endothermic peak was observed at 240 °C, which is most likely a crystalline melting point (Tm).

(Color online). DSC thermograms of PAAm and HQ–PAAm ([BQ]/[AAm] = 5).
The thermal stability of both PAAm and its modified HQx–PAAm (x = 0.58) is elucidated by their thermograms in Fig. 5. An overall trend was that the modified polymer was by far more thermally stable than the unmodified one. As for HQ–PAAm, several degradation stages occurred: the first stage was small (∼5% weight loss) and occurred from 100–220 °C, followed by a second stage (∼15% weight loss) from 250–350 °C; a third stage occurred with ∼25% weight loss at approximately 400 °C, and the last stage was observed with 50% weight loss at 800 °C. Their maximum mass losses occurred at 393 and 795 °C. The thermogram of the virgin PAAm indicated that a weight loss of approximately 80% occurred at 400 °C and that it is entirely decomposed at nearly 1000 °C.
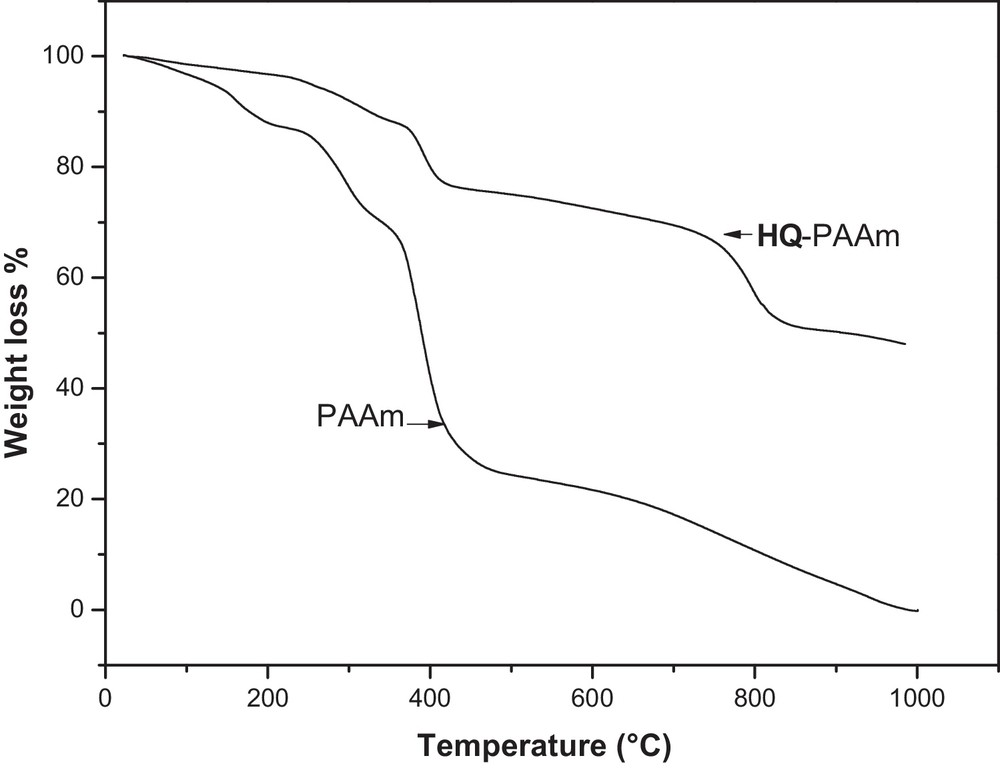
TGA curves of PAAm and HQ–PAAm ([BQ]/[AAm] = 5).
Fig. 6 shows the XRD spectra of PAAm and HQ–PAAm. As shown, the diagram profiles indicate an amorphous morphology for both, with broad bands appearing at 2θ ≈ 24°. However, a decrease in the amorphous state and a slight degree of crystallinity were engendered upon this modification as new sharper and less intense bands emerged at 2θ of approximately 22, 27, 30, 39, and 43°.

(Color online). XRD spectra of PAAm and HQ–PAAm ([BQ]/[AAm] = 5).
Analysis of the polymers by SEM (Fig. 7) clearly confirmed the extent of modification. The morphology of PAAm consists of cavities with varying dimensions, and that of HQ–PAAm is filled and less porous.

SEM images: a: PAAm, ×230; b: HQ–PAAm ([BQ]/[AAm] = 5), magnification: ×230; c: HQ–PAAm ([BQ]/[AAm] = 5), magnification: ×1600.
3.1.1 Effects of time and of the [BQ]/[AAm] ratio on the degree of substitution
The degree of modification was found to increase with time up to approximately 5 h, beyond which the effect was slight; substitutions of approximately 22% and 60% occurred at 30 min and 6 h, respectively, for a [BQ]/[AAm] molar ratio of 5. The effect of the [modifier]/[AAm] molar ratio on the extent of substitution of the PAAm modification was assessed at 70–80 °C for two reaction times, 3 h and 6 h (Fig. 8). The degree of modification was estimated by UV absorbance of the modified polymer using a calibration curve set by measuring the absorbances of dilute hydroquinone solutions at λmax = 288 nm. As shown in Fig. 9, the variation in the degree of modification increased with increasing [BQ]/[AAm], and a high degree of substitution of nearly 39% was obtained with a molar ratio of 5. Additionally, increasing the reaction time from 3 h to 6 h improved the extent of substitution to approximately 60.02%.

Variation of the substitution degree, S(%), as a function of the [BQ]/[AAm] molar ratio: a: reaction time = 3 h; b: reaction time = 6 h.
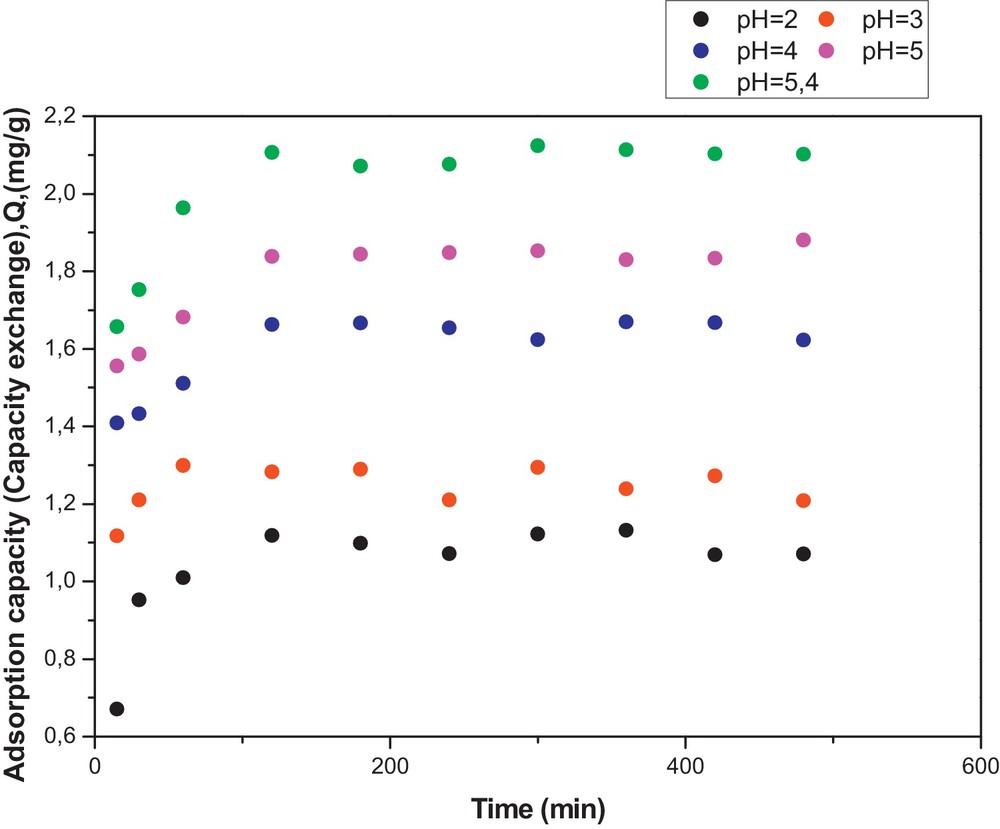
(Color online). Adsorption capacity of PAAm towards Cu(II) as function of time and pH. Temperature: 25 °C, [NaNO3] = 0.1 M/L (ionic strength).
3.2 Metallic adsorption–desorption study
Acrylic polymers are versatile metal-chelating resins [26,27]. Our goal in incorporating HQ motifs onto the PAAm backbone was to examine their effect on the metal-binding capacity of PAAm.
PAAm and HQ–PAAm adsorbents were made by cross-linking their corresponding soluble polymers and by applying the oxidative decarboxylation conditions of Minisci, as outlined in Schemes 2 and 3. The HQ–PAAm used for the adsorption of the metallic ions was the one modified with a [BQ]/[AAm] molar ratio of 5, corresponding to a degree of substitution of ∼58.49%. The differing results for copper ion adsorption by PAAm and HQ–PAAm are illustrated in Figs. 9–13. The effects of pH, ionic strength, time, and temperature on the metallic adsorption capacities of the resins were also assessed.
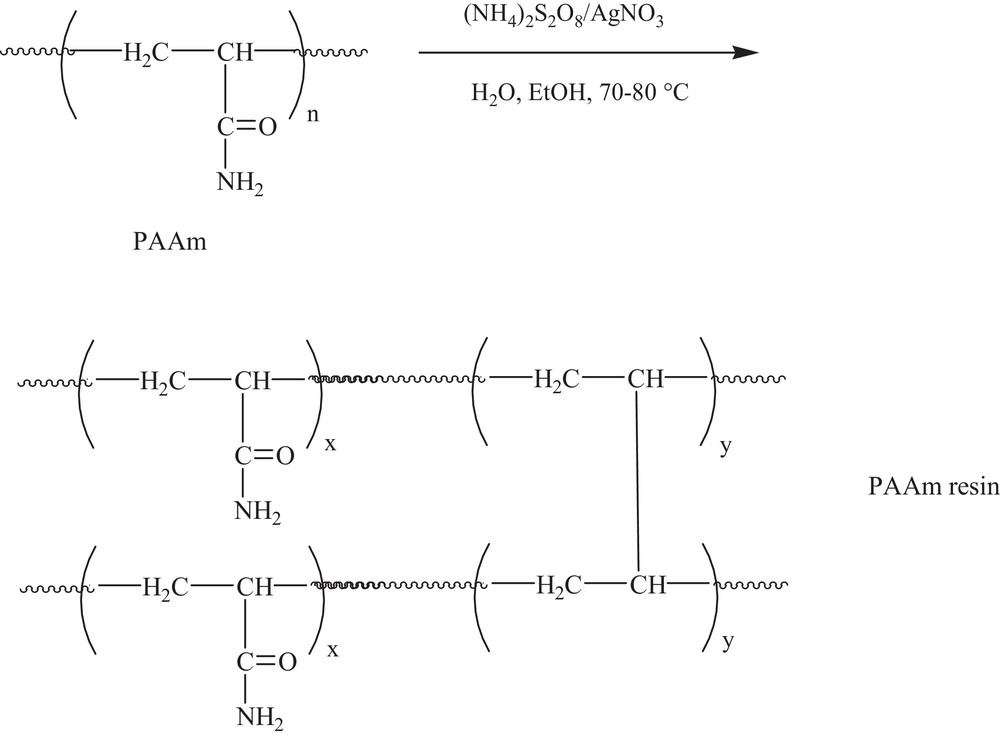
PAAm resin via cross-linking under Minisci's conditions, as shown in Scheme 1.

HQ–PAAm resin via cross-linking under Minisci's conditions, as shown in Scheme 1.
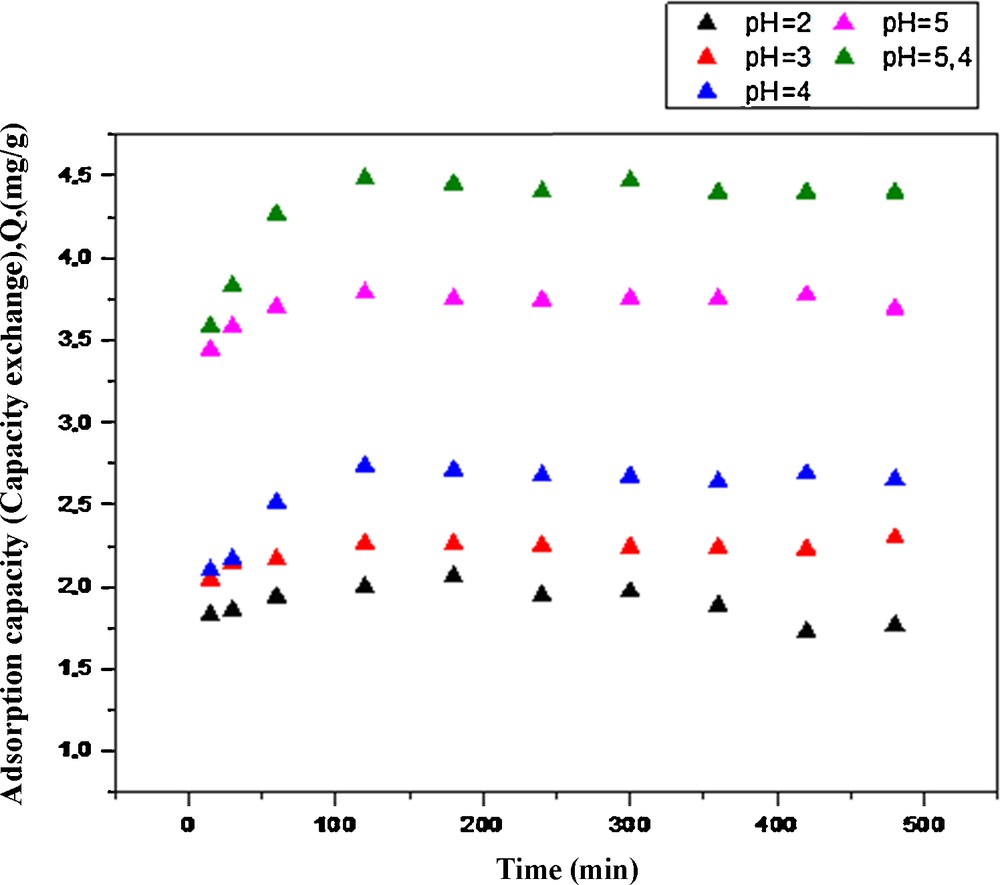
(Color online). Adsorption capacity of HQ–PAAm ([BQ]/[AAm] = 5) towards Cu(II) as a function of time and pH. Temperature: 25 °C, [NaNO3] = 0.1 M/L (ionic strength).

Adsorption capacity of HQ–PAAm ([BQ]/[AAm] = 5) towards Cu(II) as a function of time and ionic strength. Temperature: 25 °C, pH = 5.4.

Adsorption capacity of PAAm and HQ–PAAm ([BQ]/[AAm] = 5) towards Cu(II) as a function of time and temperature. pH = 5.4; [NaNO3] = 0.01 M/L (ionic strength), Temperature: 25 °C.

Adsorption capacity of PAAm and HQ–PAAm ([BQ]/[AAm] = 5) towards Pb(II), Cu(II) and Cd(II) as a function of time. pH = 5.4; [NaNO3] = 0.01 M/L (ionic strength).
An overall trend was that the adsorption capacities of the PAAm and HQ–PAAm resins increased with respect to copper (II) with increasing pH and increasing time up to 2 h. Moreover, the pH of the solution remained almost unchanged during the adsorption tests, suggesting a buffering capacity of the complexes formed. This experiment was limited to the indicated pHs, because copper hydroxide precipitates at a pH higher than 5.4, which would then interfere with the actual results. At the highest pH (5.4), copper uptake by the PAAm and HQ–PAAm resins was nearly 2.10 and 4.47 mg/g, respectively; that is, an almost twofold increase in the adsorption capacity by the modified resin with respect to the unmodified one. Such a difference in adsorption capacity could be an effect of the hydroquinone moiety. The impact of the pH parameter on the removal of metallic ions from solutions is clear because it affects the surface charge of the sorbent. That is, a higher pH would favor the tendency of negative charge formation on the sorbent, leading to an increase in the electrostatic attraction between positively charged metal ions and the negatively charged sorbent, thus increasing metallic sorption. Notably, the adsorption profile for the PAAm resin appeared to be indented beyond the two-hour adsorption period, suggesting the occurrence of a slight adsorption–desorption cycle, whereas that for HQ–PAAm resin leveled off smoothly as previously observed [21]. As to the effect of ionic strength (Fig. 11), the results indicated that higher ionic strength concentrations disfavored Cu(II) adsorption, suggesting that Na+ had some affinity towards the adsorption sites; for example, at an ionic strength of 0.01 g/mol, the adsorption capacity was 5.01 mg/g, and at 0.5 g/mol, a 50-fold increase, the adsorption capacity dropped to 3.36 mg/g.
Fig. 12 illustrates the impact of temperature along with time on the Cu(II)-uptake capacity of the resins. In general, the adsorption capacity increased with temperature. Indeed, for the PAAm resin, an increase in adsorption from 2.78 mg/g at 25 °C to 4.51 mg/g and to 5.95 mg/g was measured at 35 and 45 °C, respectively. Likewise, for the HQ–PAAm resin, an increase in capacity from 5.01 mg/g to 6.89 and 7.20 mg/g occurred at the same temperatures. It can be noted that, above 45 °C, the adsorption capacity of the latter resin was not further enhanced.
Tables 2 and 3 list the results of the sorption of the Cu(II) copper ion upon varying the adsorbing resin mass and the initial copper concentration, respectively. With regard to the former effect, 99.45 and 181.70% enhancements of the exchange capacity of PAAm and of HQ–PAAm resin, respectively, were observed in optimal conditions (Table 2). Raising the initial concentration of copper nitrate by ten-fold improved the metal ion uptakes of the resins (Table 3) by approximately 96 and 26% for the resins, respectively.
Sorption of copper ion Cu(II) at different sorbent masses.
Sorbent mass (g) | Sorption of Cu2+ (mg/g) | |
PAAm | HQ–PAAm* | |
0.05 | 1.84 | 2.90 |
0.10 | 2.78 | 5.01 |
0.20 | 3.67 | 8.17 |
* HQ–PAAm from [BQ]/[AAm] = 5; the degree of substitution was 58.49%.
Sorption of copper ion Cu(II) at different initial copper ion concentrations.
Initial concentration of copper nitrate, C0 (mg/L) | Sorption of Cu 2+ (mg/g) | |
PAAm | HQ–PAAm* | |
10 | 1.69 | 4.16 |
50 | 2.78 | 5.01 |
100 | 3.31 | 5.67 |
* HQ–PAAm from [BQ]/[AAm] = 5; the degree of substitution was 58.49%.
Adsorption of Pb(II) and Cd(II) by the resins was tested and compared with that of Cu(II) under the same optimal conditions. The adsorption capacities of the resins towards these metal ions are depicted in Fig. 13. The maximum adsorptions for Pb(II) and Cd(II) were as follows: 3.16 and 1.81 mg/g, respectively, for the PAAm resin, and 5.91 and 4.36 mg/g, respectively, for the HQ–PAAm resin. As given in Table 4, these adsorption capacities are within the range for dihydroxybenzene-bearing polymers and lower than those for tetrahydroxybenzene-functionalized polyacrylics. Under the actual experimental conditions, the exchange capacity follows the following order: Pb(II) > Cu(II) > Cd(II). This finding is in agreement with the order of binding constants for metallic ions with catechol derivatives [28]. This metallic uptake order was also observed by Moulay et al. [20,21] and also when bentonite and clinoptilolite were employed as clay adsorbents [29].
Adsorption capacity (mg/g) of some polyhydroxybenzene-functionalized resins towards Cu(II), Cd(II), and Pb(II).
Polymer | Functionalizing group | Cu(II) (mg/g) | Cd(II) (mg/g) | Pb(II) (mg/g) | Reference |
Cellulose | Catechol | 11.83 | 13.00 | 21.5 | [30]* |
Amberlite XAD-4 | Catechol | 5.69 | 2.89 | / | [31]* |
Poly(vinylcatechol-co-divinylbenzene | Catechol | 6.44 | 9.10 | / | [32]* |
Poly(acrylic acid) | Benzene-1,2,4,5-tetrol | 19.54 | 14.90 | 22.16 | [20] |
Polyacrylamide | Benzene-1,2,4,5-tetrol | 18.87 | 17.58 | 20.74 | [21] |
Polyacrylamide | Hydroquinone | 5.01 | 4.36 | 5.91 | Present work |
* The mentioned values were computed from the results given in the corresponding articles.
Desorption of metal-adsorbed resins was accomplished by treatment with 0.1 N nitric acid at 25 °C. The results of adsorption–desorption of ions by the resins are listed in Table 5. Interestingly, the capacity exchanges of the resins were retained after three adsorption–desorption cycles for Cu(II), Pb(II), and Cd(II) ions. The extents of desorption of these metallic ions from the PAAm and HQ–PAAm resins were significantly high and exceeded 97%. The performance of the regenerated resins through adsorption–desorption cycles was very high for all the metallic ions tested.
Results of adsorption–desorption of Cu(II), Pb(II) and Cd(II) by PAAm and HQ–PAAm resins.
Resin | Cycle | Amount of ion adsorbed (mg) | Amount of ion desorbed (mg) | Desorption extent R (%) | ||||||
Cu2+ | Pb2+ | Cd2+ | Cu2+ | Pb2+ | Cd2+ | Cu2+ | Pb2+ | Cd2+ | ||
PAAm | 1 | 3.70 | 4.89 | 2.93 | 3.68 | 4.87 | 2.92 | 99.41 | 99.51 | 99.74 |
2 | 3.67 | 4.82 | 2.85 | 3.65 | 4.79 | 2.84 | 99.39 | 99.48 | 99.49 | |
3 | 3.66 | 4.70 | 2.82 | 3.64 | 4.67 | 2.80 | 99.24 | 99.44 | 99.31 | |
HQ–PAAm* | 1 | 8.17 | 8.71 | 8.71 | 8.04 | 8.59 | 7.18 | 98.33 | 98.58 | 98.91 |
2 | 8.16 | 8.65 | 8.65 | 7.96 | 8.46 | 7.09 | 97.54 | 97.82 | 98.84 | |
3 | 8.13 | 8.62 | 8.62 | 7.89 | 8.40 | 7.01 | 97.08 | 97.41 | 98.13 |
* HQ–PAAm from [BQ]/[AAm] = 5; the degree of substitution was 58.49%.
4 Conclusions
The application of the oxidative decarboxylation method of Minisci to the chemical modification of polyacrylamide is successful, but with concomitant degradation. Minisci's conditions could profitably promote the formation of polyacrylamide resins as sorbents. Hydroquinone-grafted polyacrylamide is better suited than the virgin polyacrylamide as far as metallic adsorption is concerned. The adsorption–desorption results suggest that hydroquinone–polyacrylamide should be used as a chelating cation exchange resin with good adsorption–desorption repeatability.
Acknowledgements
T. Dintzer is thanked for his assistance with the SEM measurements. Part of this work was supported financially by the Minister of Defense of Algeria, to which N. Bensacia is deeply grateful. I. Fechete would like to thank CNRS (France) for financial support.