1 Introduction
The unique characteristics of type I collagen make it the most investigated natural polymer as drug delivery substrate, including dressings for wound healing. Thus, besides full biodegradability, weak antigenicity and restorability that make it a primary source in the biomedical application [1,2], it interferes in all the stages of normal healing [3]: haemostasis, formation of granular tissue [4], fibroblasts proliferation and reepitelization, provides resistance and integrity to the wound [5]. Being a protein, it cannot promote by itself the healing process, serving as substrate for development of bacteria. But associated with antimicrobials and applied topically, it functions as delivery system, controlling the wound infection [6–8]. Moreover, such dressings reduce the levels of protease, cytokines and free radicals [9].
Increasing in prevalence of microbial resistance to conventional antibiotics focused the attention upon the natural antimicrobial [10], especially phytochemicals used traditionally to treat infected wounds [11]. Many of the secondary metabolites have antibacterial properties, serving to defend plants from microbial infection [12]. They can modulate or modify bacterial resistance and potentate the activity of antibiotics/antiseptics [13,14].
Vegetable tannins are polyphenols occurred in a high variety of plants [15]. Tannic acid (gallotannin), TA, the main representative of hydrolysable tannins, has antiviral, antimicrobial and antibacterial properties (its minimal inhibitory concentration – MIC ranges between 150 and 1000 mg/L, depending on the microbial strains) [16–18]. At the same time, TA exhibits some other beneficial effects: antimutagenic and antigenic activity [19] induces apoptosis in animal tumour cells as resulted from both in vitro and in vivo tests [20–22], is implied in hyaluronidase system, has antioxidant action [21] and inhibitory effect on Clostridium histolyticum collagenase activity, preventing the degradation of extracellular matrix components [23]. It is the tannin having the highest affinity for collagen [24,25].
Chlorhexidine digluconate (1,1′-hexamethylenebis[5-(p-chlorophenyl)-biguanid]di-d-gluconate), CHDG, one of the most used antimicrobials in clinical practice for skin antisepsis [26], is potent also against mutant streptococci [27,28]. Its efficacy is reduced by pH decreasing and the presence of organic matter [29], but can be improved by phytochemicals, some of them transforming CHDG in vivo from bacteriostatic into bactericide [11,14,30]. TA might be one of the biologically active secondary metabolite able to increase the antimicrobial activity of CHDG.
The objective of the present paper is the preparation of 1.1% collagen hydrogels containing all the combinations between 1.82, 4.55 and 9.09% CHDG and 5, 10 and 15% TA and their structural and rheological characterization to evaluate the preservation degree of native conformation of collagen and also to establish whether these systems are suitable to be applied topically as dressings for wound healing and/or for obtaining porous matrices or films.
2 Experimental
2.1 Materials
Collagen hydrogel 2.54%, pH 3.21 was provided by the National Research-Development Institute for Textiles and Leather, Division Leather and Footwear Research Institute–NRDITL-LFRI, Romania; collagen – as 1% acetic solution –was prepared in laboratory after its extraction from calf skin and subsequent purifications; TA was acquired from Sigma-Aldrich and CHDG (20% aqueous solution, by weight) from Fagron (Germany) and were used as received. A solution of 1M NaOH (analytical grade, Reactivul–Bucharest, Romania) was also used to adjust the final pH of the collagen-based systems.
2.2 Preparation of collagen hydrogels containing TA and CHDG
Three series of 1.1% collagen hydrogels having pH 3.8 and compositions inserted in Table 1 were prepared by adding proper amounts of TA and CHDG solutions under manual stirring to obtain the desired concentrations as described elsewhere [31]. To maintain the overall pH of the systems at 3.8 during collagen-based hydrogels preparation, a solution of 1 M NaOH was used. A series of collagen hydrogels containing TA for comparison was also prepared. All the hydrogels were equilibrated by keeping them at 4 °C for 24 h.
Viscosities at zero shear rate and angular frequency of 1 rad·s−1 for collagen hydrogels obtained by rotational viscometry and dynamic rheology measurements, respectively.
CHDG [%] | TA [%] | η0 [Pas] | (η)1 rad·s–1 [Pas] |
0 | 9.8 | 19.1 | |
5 | 13.9 | 39.8 | |
0 | 10 | 21.5 | 56.1 |
15 | 19.2 | 58.2 | |
5 | 10.1 | 41.6 | |
1.82 | 10 | 15.2 | 43.4 |
15 | 20.7 | 52.8 | |
5 | 15.5 | 41.0 | |
4.55 | 10 | 16.7 | 81.2 |
15 | 11.9 | 66.7 | |
5 | 21.2 | 66.0 | |
9.09 | 10 | 14.8 | 76.9 |
15 | 9.1 | 158.6 |
2.3 Structural characterization of collagen
Collagen from hydrogels was characterized by FT–IR using an ABB MB3000 MID-IR spectrometer equipped with a DTGS detector and Horizon software. Data were acquired by ATR technique using a PIKE 45 degree ZnSe trough plate with volatile cover Horizontal ATR. All the spectra were corrected for ATR effect and transformed into absorption ones. Each spectrum is the average of 32 scans, with a resolution of 4 cm−1.
UV–CD spectra of hydrogels were recorded employing a Jasco J-810 spectropolarimeter using a 0.2-cm path length square Suprasil cuvette and the following operating parameters: wavelength range 250–190 nm; scanning speed 50 nm/min with 0.2-nm pitch and 2-s response time, room temperature (23 °C), average number of spectra accumulation 4, slit width 400 μm, continuous feeding of measuring compartment with high purity nitrogen (99.9995%) to suppress oxygen absorption.
2.4 Rotational viscometry and dynamic rheological measurements
Shear rheological behaviour was investigated at 23 ± 0.1 °C by use of a rotational viscometer Haake VT 550 equipped with MV1 sensor system for medium viscosity and RheoWin 4 Thermo Fischer Scientific software for data acquisition and processing.
Oscillatory measurements were performed at room temperature (23 °C) using a Micro Fourier Transform Rheometer MRF 2100, GBC-Australia, working under squeezing flow, with the operating parameters set as follows: angular frequency 0–1000 rad·s−1, 280 discrete frequencies analysed simultaneously in range by a step of 0.5 s−1, 30 spectra consequently acquired for each sample, gap between the upper and bottom plates 400 μm, displacement amplitude 0.03 μm to fall into the linear viscoelastic domain. The very small amplitude of pseudorandom squeezing motion allows a continuous monitoring of force transmitted through the viscoelastic sample to the force sensor (bottom plate). Storage and loss moduli at each individual frequency are obtained by a Fourier transform processing.
3 Results and discussion
To keep collagen's advantages in wound healing, its specific triple helix conformation has to be preserved [32–34]. This makes the assessment of its conformation the first step in the preparation of collagen-based biomaterials.
The presence of triple helical conformation in collagen hydrogels has been evaluated by the usual methods applied to collagen: FT–IR [35–38] and UV–CD [39].
The FT–IR spectrum of collagen hydrogel, presented in Fig. 1, exhibits the band amide I at 1655 cm−1 with a shoulder at 1630 cm−1, amide II at 1556 and 1523 cm−1, CH2 wagging at 1454 cm−1, amide III at 1240 and 1205 cm−1, and the bands assigned to C-O stretching at 1082 and 1041 cm−1, according to the literature data [38,40–46]. The difference between the wavenumbers of amide I and II bands, indicating the absence of denatured collagen when the value is lower than 100 cm−1, is 99 cm−1, which demonstrates the integrity of the native triple helical conformation of collagen in the hydrogel.
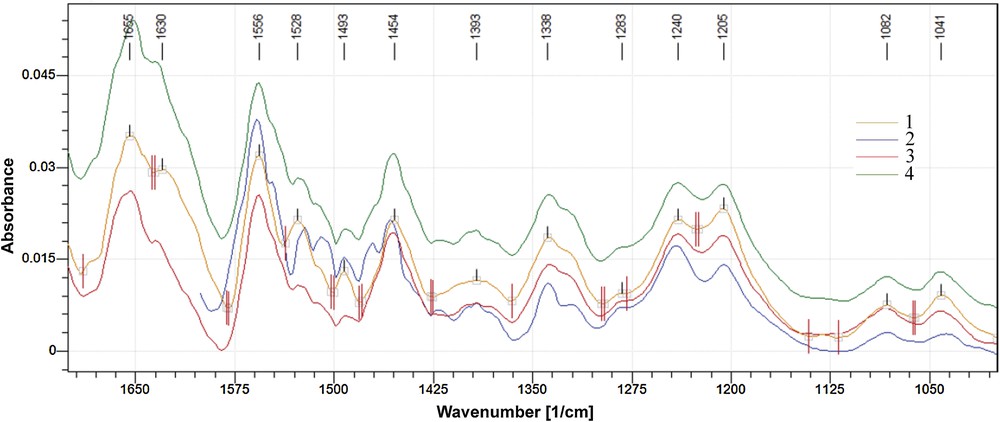
(Colour online). Superposed FT–IR spectra of reference hydrogel (1, collagen only) and of collagen hydrogels containing: 5 (2), 10 (3) and 15% (4) TA.
Fig. 1 shows that the FT–IR spectra of hydrogels containing TA exhibit, on the whole, the same pattern as the reference does, excepting the band amide II which is different: the intensity of 1528 cm−1 band decreases slightly and a shoulder appears at 1545 cm−1. This can be due to partially removing of water by TA and forming of new hydrogen bonds with collagen. Therefore, the triple helix is not affected and cross-linking occurs with preserving the native collagen structure.
The FT–IR spectra of hydrogels containing 1.82% CHDG and 5, 10 and 15% TA look very similar to those in Fig. 1 and not with those containing CHDG [31]. This means that TA-collagen interaction is stronger than CHDG-collagen one. The FT–IR spectra of hydrogels containing CHDG also show a very weak interaction between components and practically no effect on triple helix conformation [31]. TA and CHDG cause a slight increase of the intensity band at 1528 cm−1, assigned to hydrogen bonds forming between TA and CHDG, and vanishing of the shoulder at 1545 cm−1 with increasing TA concentration. The difference between the corresponding wavenumbers of amide I and II bands ranges between 95 and 100 cm−1, with the highest value for 15% TA system, which means that the denatured collagen is absent.
The FT–IR spectra of hydrogels containing 4.55% CHDG and the above amounts of TA exhibit almost the same pattern of bands as the preceding ones. Thus, the increasing of CHDG amount does not modify significantly the interactions within the system collagen-TA-CHDG. An exception is the band amide I in the hydrogel containing 5% TA, shifted towards higher wavenumbers by 8 cm−1. This can be due to the stretching vibration of the peptide carbonyl group, which is very sensitive to hydrogen bonding. Also, the increasing of intensity of band at 1528 cm−1 with increasing TA concentration is a little higher, which support the above assignment of the band (hydrogen bonds forming between TA and CHDG). The difference (νamide I – νamide II) is 103 cm−1, very close to 100 cm−1, which means that the denatured collagen is practically absent from this series of hydrogels.
The FT–IR spectra of hydrogels with 9.09% CHDG and the three amounts of TA are more particular and they are shown in Fig. 2.
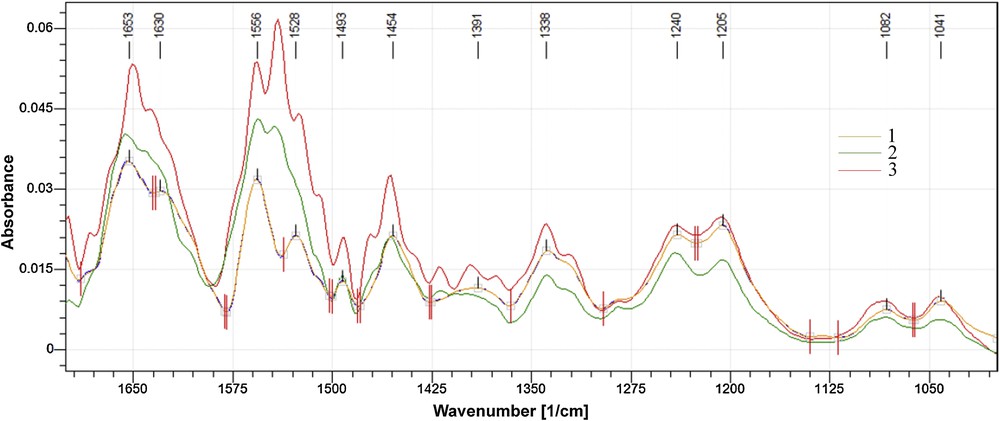
(Colour online). Superposed FT–IR spectra of collagen hydrogels containing 9.09% CHDG and: 5 (1), 10 (2) and 15% (3) TA.
The bands amide I and III preserve both the shape and position, while amide II, at 1556 cm−1, splits and a new band appears at 1541 cm−1 starting with 10% TA. As the intensity of the new band is a little lower than that at 1556 cm−1 for the hydrogel containing 10% TA, it becomes more intense for 15%. The new band could be assigned to the interactions of phenolic –OH of TA with secondary amine groups from chlorhexidine structure, placed on each part of the hexamethylen bridge [47,48]. But this becomes evident only when the two components have higher concentrations. Moreover, differences could also be found in the aspect of the CH2 wagging band: it has a weak shoulder at 1470 cm−1 when TA concentration is 10%, which increases in intensity for 15%. This could be assigned to the hydrophobic interactions between hexamethylene bridges and hydrophobic groups of TA, which intensify when the concentration of the two components increases. The differences (νamide I – νamide II) range between 95 and 100 cm−1, which practically demonstrates the lack of denatured collagen even in this hydrogel series. Therefore, the collagen retains its native conformation in all the prepared hydrogels and it is proper to be used as dressings.
On the other hand, it has been well documented that a pronounced negative minimum around 200 nm, a weak positive maximum at 220–225 nm and a cross point (at which the ellipticity is cancelled) at about 212 nm are the features of the UV–CD spectrum of native collagen [49–51]. At the same time, the ratio of absolute values of intensities of positive peak over the negative one, Rpn, is a measure of the degree of helicity of collagen [52] and ranges between 0.12 and 0.15 for aqueous dilute collagen solutions (below 0.2%) [53,54]. Partial denaturation of collagen results in a red shift of the negative band, decreasing in intensity of the positive one and Rpn and a red shift of the cross point [35,53].
The UV–CD spectrum of 1.1% collagen hydrogel is shown in Fig. 3, together with those of hydrogels with TA concentrations of 5, 10 and 15%, respectively.
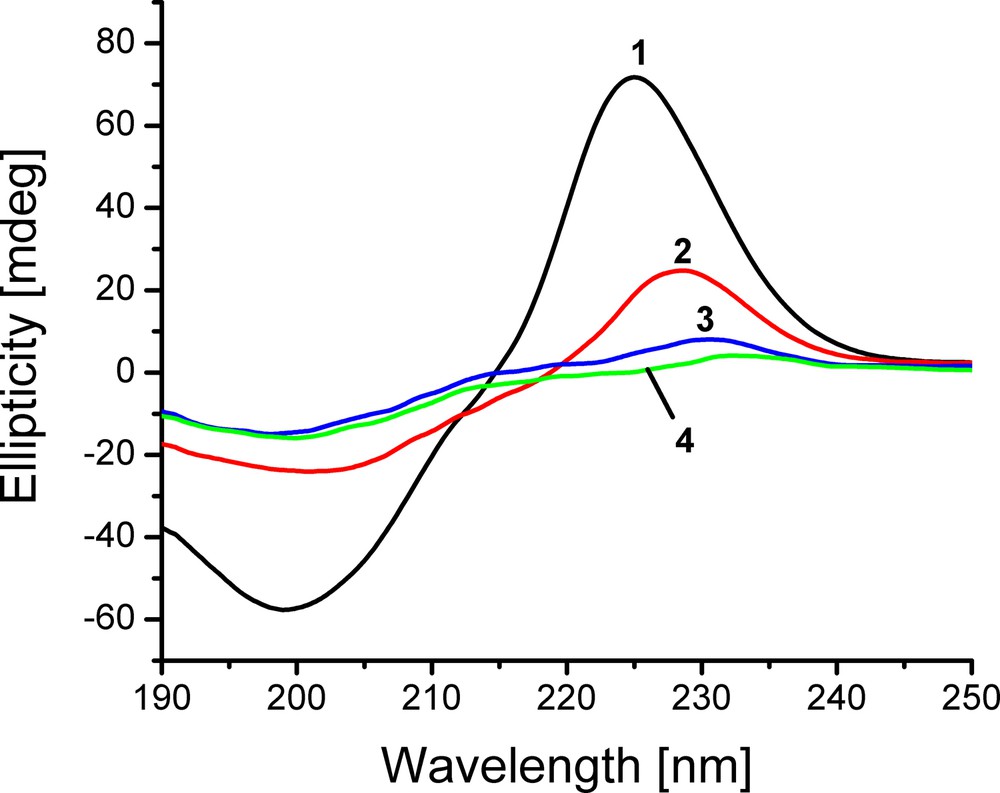
(Colour online). UV–CD spectra of collagen hydrogels: reference (1, collagen only) and containing 5 (2), 10 (3) and 15% (4) TA.
For collagen hydrogel with no TA, this revealed a negative peak located at 199 nm, a maximum at 225 nm and a cross point at 214.8 nm, which generally defines a set of features peculiar to the native (triple helical) conformation of collagen. However, Rpn value of 1.24 is much higher than that found in aqueous dilute solutions of collagen [53,54]. Such a result, as it will be seen in the following, formally derived from significantly descending in absolute intensity of the negative peak, was in fact induced by the higher concentration of collagen in hydrogel.
To establish the dependence of intensities of negative and positive peaks on collagen concentration, the UV–CD spectra of collagen in solutions of different concentrations were recorded and plotted in Fig. 4. Thus, increasing collagen concentration has given rise to the following effects: intensifying the positive peaks while the wavelengths for peak maxima were preserved; shifting the peak minima towards higher wavelengths and dramatically diminishing the negative peaks within the collagen concentration range 0.16–0.67%; ascending Rpn from 0.1 to 1.9; keeping the cross points at the same wavelength value: 214.2 nm. Based on these findings, it has become clear that such a behaviour observed in CD spectra under the influence of collagen concentration does not imply either partial or entire collagen denaturation at all.

(Colour online). UV–CD spectra of collagen of specified concentrations in 0,1 M aqueous acetic acid solutions.
At the same time, Fig. 3 shows that the positive peaks in UV–CD spectra for the collagen from hydrogels decrease with increasing TA concentration whilst the negative peaks and cross points do not vary regularly in their size and location, respectively. The Rpn values decrease, becoming 1.03, 0.53 and 0.26, respectively, for 5, 10 and 15% TA. All these changes seem to outline a process of partial collagen denaturation [35,55]. It could be thought that hydrogels with TA contain partially or entirely denatured collagen, with a denaturation extent increasing with TA amount. However, taking into account the results for concentrated collagen solutions, on one hand, and the differences between the wavenumbers corresponding to the amide I and II bands for collagen hydrogels, on the other hand, one can conclude that all the hydrogels are devoid of denatured collagen. At the same time, it was established that the tanning process does not alter the native collagen conformation [52].
The UV–CD spectra of hydrogels containing CHDG and TA are shown in Fig. 5a.
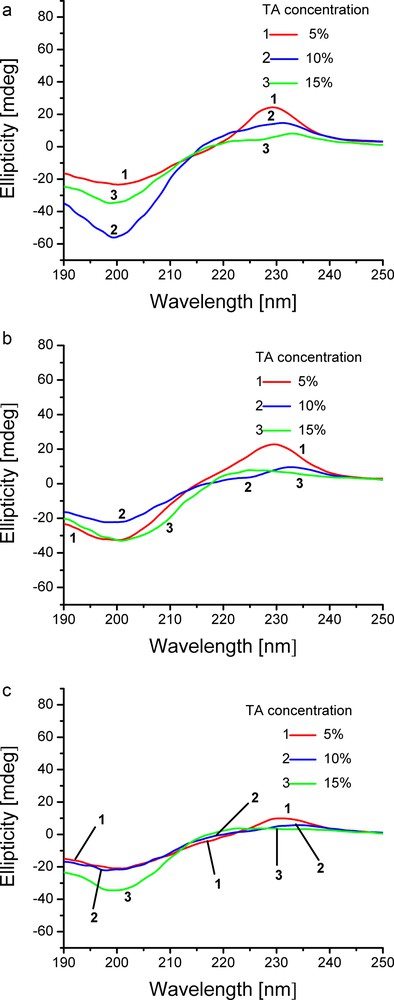
(Colour online). UV–CD spectra of collagen hydrogels containing: (a) 1.82%, (b) 4.55% and (c) 9.09% CHDG at the specified amounts of TA.
When hydrogels contain 1.82% CHDG, important decreases of positive peaks and slight displacement of peak maxima towards higher wavelengths as TA amount increases took place (from 229 nm for 5% TA to 231 nm for 10% TA and to 233 nm for 15% TA), the peak minima were slightly displaced towards lower wavelength (200 nm for 5% TA, 199 nm for both 10 and 15% TA), whilst the negative peak size varied irregularly. Cross points underwent a slight red shift and Rpn changed from 1.04 to 0.26 and 0.23 as TA concentration increased from 5 to 10 and 15%, respectively. This behaviour, by comparison to the results obtained for collagen solutions and collagen-TA hydrogels, was brought about by the high collagen concentration.
Another factor affecting the UV–CD spectra is the turbidity of the measured systems. Both collagen solutions and hydrogels are pretty turbid systems. Their turbidity generally increases with collagen concentration and therefore, a point (wavelength value) at which the light absorption becomes lower than light scattering may be reached, which alters the shape of UV–CD spectrum. At the same time, TA increases the hydrogels turbidity and CHDG makes it even more prominent. The most turbid hydrogel was that containing the highest amounts of both compounds. Moreover, an incipient phase separation could be seen, which reduced the sample homogeneity and distorted the spectra (Fig. 5).
A concentration of 4.55% CHDG, Fig. 5b, brought about the same tendency regarding the variation of both cross point and Rpn values on TA concentration. Thus, the cross points exhibited a slight red shift while the Rpn values descended from 0.7 to 0.43 and 0.24 as increasing TA.
The collagen-TA hydrogels with the maximum amount of CHDG, Fig. 5c, displayed a similar bias only for Rpn values (0.47, 0.27 and 0.11 as TA increased). Instead, a conspicuous behaviour that needs further investigations was observed as regards peak and cross point displacement. Therefore, the peak maxima showed a red shift and the peak minima a blue shift, while the cross points were displaced towards lower wavelengths.
Again, based on the results evidenced in studying collagen solutions (Rpn decreases as collagen concentration and overall turbidity increase) and the FT–IR data on collagen-TA and collagen-TA-CHDG hydrogels, we believe that the simultaneous presence of both components (TA and CHDG) into the collagen hydrogels does not alter practically the native collagen structure at least for the concentration ranges investigated and hereby the hydrogels can be used as biomaterials. The distortions of CD spectra were induced by the high collagen concentration and the tendency of incipient phase separation which increased turbidity of hydrogels, both of them progressively intensifying as concentrations of components increase.
Rheology, as an experimental approach in studying the relationship between structure and flow properties of a material and also between the magnitude of a strain and the force that actuates it, allows the proper designing and/or formulation of materials, predicts the way of applying onto a proper substrate and the complex behaviour a material undergoes during its processing. The most important properties of a hydrogel are the flow and gel properties that can be adequately provided by the rotational-transient test and dynamic rheology, respectively.
Rotational viscometry applied to collagen hydrogels was performed at low shear rates, , to prevent the destroying of gel structure. The rheograms of the reference hydrogel of those containing TA and 4.55% CHDG and TA are plotted in Fig. 6a and b.
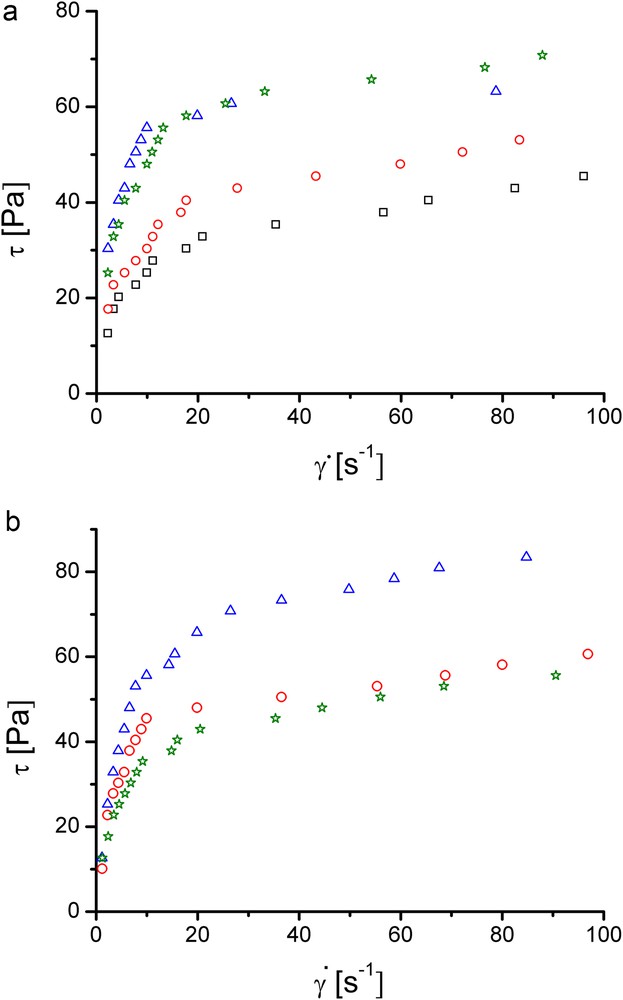
(Colour online). Rheograms of collagen hydrogels: (a) containing TA 0% (squares), 5% (circles), 10% (triangles) and 15% (stars); (b) with 4.55% CHDG and TA concentration of 5% (circles), 10% (triangles) and 15% (stars).
All the rheograms in Fig. 6a and b show common characteristics: an overall pseudoplastic behaviour that seems to exhibit two distinct regions of ideal plastic behaviour - one at low shear rates and the other one at shear rates exceeding 20 s−1; yield stresses almost zero Pa; gel structure affected by a shear rate of ca. 10 s−1.
The cross-linking effect of TA on the collagen hydrogels is visible in Fig. 6a: the rheograms of hydrogels with 5 and 10% TA are placed above the reference one over the entire range of shear rates. Instead, the curve for the hydrogel containing 15% TA is almost superposed on that with 10% TA, especially at shear rates higher than about 10 s−1. This can signify that a content of 10% TA is high enough to cross-link the collagen from hydrogel and the excess may be detrimental, producing an incipient phase separation.
The rheograms corresponding to the collagen hydrogels containing 1.82% CHDG and TA (not graphically shown) have the same shapes as those displayed in Fig. 6a, but the values of shear stresses are lower. This can be due to the interaction of TA with CHDG, which form associates by hydrogen bonding, reducing therefore the amount of TA implied in collagen cross-linking. When CHDG amount is 4.55%, the placement of rheograms (Fig. 6b) is pretty different: that of hydrogel containing 15% TA lies the lowest position, close to that for 5%, especially at shear rates higher than 20 s−1, while that of hydrogel with 10% is the uppermost curve. At the same time the last hydrogel is the most resistant to shearing, proving that this is the best CHDG-TA combination to cross-link the collagen into the hydrogel. For the collagen hydrogels containing 9.09% CHDG and various amounts of TA (results not shown), the rheograms for 5 and 10% TA are located pretty close and a bit above that of the reference, whilst that for 15% TA is placed upper, due to the higher amount of TA remained for cross-linking after interaction with CHDG.
The measure of cross-linking and gel destruction as a function of TA content is reflected by the dependences of apparent viscosities, η, on shear rates, from which the viscosities at zero shear rate, ηo, were obtained. They were determined by linearization within the shear rates range 0–10 s−1, in which the gel structure is practically unaffected, and is shown in Fig. 7 for the hydrogels containing 4.55% CHDG and TA.
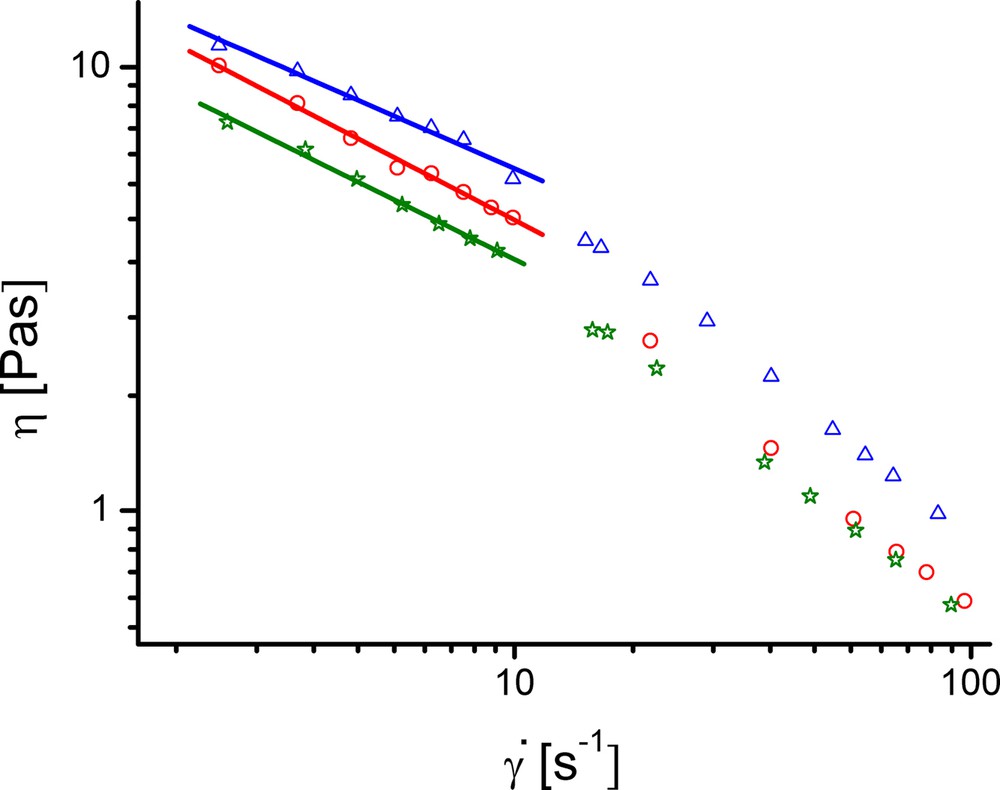
(Colour online). Dependences of η on for collagen hydrogels with 4.55% CHDG and: 5 (circles), 10 (triangles) and 15% TA (stars).
As can be seen, each dependency consists of two distinct straight lines regions crossing at ca. 10 s−1. This particular value of shear rate corresponds to the onset of the collagen gel destruction. The same behaviour and value of critical shear rate are valid for all the other collagen hydrogels. The zero shear rate viscosities estimated from the linear dependencies in a logarithmic scale, based on the shear rates values less than 10 s−1, are given in Table 1.
The results show an increase of zero shear rate viscosities with increasing TA amount in the absence of CHDG, excepting the hydrogel with 15% TA. In this case, the higher amount of TA exceeds that required for cross-linking, that hereby causes an incipient phase separation. In the presence of 1.82% CHDG, zero shear rate viscosity increases with TA amounts, but the values are lower than those of the corresponding hydrogels containing no CHDG. This is most likely due to the consumption of a part of TA by interaction with CHDG that may lead to reducing of hydrogel cross-linking. However, the ascending tendency of zero shear rate viscosity values as a function of TA content was kept even for the hydrogel containing 15% TA. This is the evidence that the total amount of TA (implied both in interaction with CHDG and cross-linking the hydrogel) is appropriately distributed between the two types of interactions, so that only the hydrogel cross-linking occurs, not phase separation at all. When the amount of CHDG is 4.55%, the hydrogel containing 10% TA, the most resistant to shearing, has the highest zero shear rate viscosity. Increasing CHDG concentration at 9.09%, the zero shear rate viscosities of collagen hydrogels decrease with TA amount, which demonstrate that not only TA plays an important role in hydrogel cross-linking, but also CHDG. On the other hand, TA-CHDG interactions, depending on their ratio and CHDG amount, may induce a lower viscosity to the collagen hydrogels. Thus, rotational viscometry method suggests forming of associates between TA and CHDG, especially when both components had high concentrations and non-homogeneities (as a result of incipient phase separations) were observed.
Measurement of storage, G’, and loss, G“, moduli at low deformation amplitude, to fall into the linear viscoelastic domain, allowed the determination of elastic and viscous contribution to the hydrogels viscoelasticity. Change in storage and loss moduli on angular frequency, ω, for chlorhexidine-free collagen hydrogels with different amounts of tannic acid, on one hand, and for collagen-TA hydrogels having the same content of 4.55% CHDG, on the other hand, is displayed in Fig. 8.
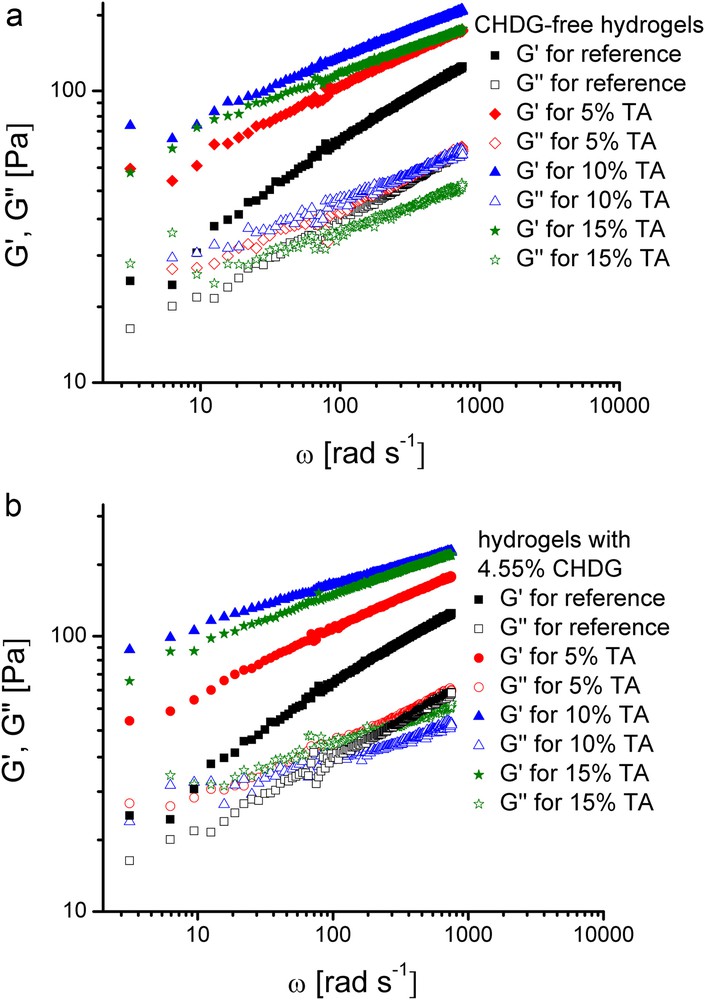
(Colour online). Evolution of G’ and G” moduli as a function of angular frequency for the inset collagen hydrogels.
The gel-like state is clearly revealed by the prevalence of the elastic part over the viscous one of the viscoelasticity of all these systems. Accordingly, the storage moduli are about two times higher than the loss ones. Generally, such a viscoelastic behaviour of a certain system beyond its gel point, where G’ > G” and the difference G’-G”, becomes higher as strength gel increases, is well-known both for physically [56–58] and chemically cross-linked gels [59,60]. At the same time, the overall viscoelasticity of a system may be also easily described by considering the quantity called loss tangent, defined as the ratio between loss and storage moduli [61–63]. Therefore, when a material exhibits a predominant viscous part of its viscoelasticity, the loss tangent exceeds unity. On the other hand, for a material in a gel-like state, depending on the gel strength, the loss tangent becomes less than unity. Based on this standpoint, the collagen hydrogels devoid of CHDG exhibit an increasing gel-like character as TA content ascends (the corresponding loss tangents having less than unity values obey a decreasing variation with TA amount), even though the maximum rigidity (expressed by the G’ value) was reached by the sample with 10% TA (Fig. 8a). All this behaviour can be viewed as an enhancing elasticity effect of TA on hydrogels viscoelasticity by a cross-linking process occurring between TA and collagen via hydrogen bonding. However, as TA content is large enough (15%, for example), incipient heterogeneities caused by TA led to an average rigidity (based on the G’ values) somewhat lower, but with a highest gel strength of the phase-separated domains of the system (based upon the lowest loss tangent value). On the other hand, taking into account the hydrogels containing 4.55% CHDG and various amounts of TA (Fig. 8b), a net effect of rigidity enhancing of the systems induced by the presence of CHDG could be noticed, most likely due to its possible role played in further physical cross-linking of collagen. This is in accordance to the higher G’ values compared to those obtained for the corresponding CHDG-free collagen hydrogels. Nevertheless, on the basis of loss tangent variation, the gel-like behaviour of these systems slightly diminished as TA content increased. Increasing the CHDG content to 9.09%, the collagen hydrogels exhibited an enhancing gel-like behaviour as shown by diminishing the loss tangent values, even though the systems displayed a slight phase separation at TA content of 10 and 15%, respectively (results not graphically shown). At the same time, the overall rigidity of these collagen hydrogels, expressed by G’ values, followed the same tendency as the gel strength did when TA increased. Such a particular feature of evolution of these collagen-TA-CHDG systems indirectly confirms significant interactions between TA and CHDG that, at high components concentrations, may give rise to insoluble aggregates and in turn to phase separations throughout the system. On the other hand, a pretty similar behaviour was observed in the case of TA-collagen hydrogels with 1.82% CHDG: the gel strength became higher as TA content increased from 5 to 15% (based on the loss tangent evolution), whereas the gel rigidity (considering variation of G’ on TA content) obeyed the order G’TA=10% < G’TA=5% < G’TA=15% that needs supplementary investigations to clarify the effects simultaneously exerted by these two components onto collagen hydrogel consistency as a function of their different content at constant concentration of collagen.
An important quantity obtained from dynamic rheometry and that describes quite well the gel consistency is dynamic viscosity (η*). The lower the angular frequency, the closer dynamic viscosity to the constant value unaffected by the oscillatory strain will result. Accordingly, the values of dynamic viscosities determined at the angular frequency of 1 rad·s−1 (0.16 Hz), (η)1 rad·s−1, by the linear fitting of the η*–ω dependences (in log-log plots), are listed in column 4 of Table 1. These viscosities have higher values compared to those obtained for the apparent viscosities since very small oscillatory strains, unlike the shearing ones, do not destroy the gel structure. They vary with TA and CHDG concentrations in the same fashion as those obtained from rotational viscometry data do, excepting the hydrogels containing 9.09% CHDG. In this case, the dynamic viscosities taken at 1 rad·s−1 increase with TA concentration, because the gel structure is not affected by the deformation regime and both TA and CHDG contribute to collagen cross-linking, especially by hydrogen bonding.
The foregoing experimental data together with the corresponding behaviours so observed allow us to outline a simplified interaction mechanism especially between the TA and collagen in hydrogel state. Therefore, due to molecular structure that consists of a pentagalloylglucose core esterified at every p-hydroxyl group by an additional molecule of gallic acid (Fig. 9a), tannic acid may act as a collagen cross-linker by hydrogen bonding [64–66] and hydrophobic interactions [65–67]. The high affinity of TA to interact with collagen via hydrogen bonding originates especially from the fifteen hydroxyl groups belonging to the five galloyl residues located into the outer shell of the TA structure and, on the other hand, the collagen functional groups like –COOH, –NH2, –OH or –NHCO-. Accordingly, in Fig. 9b, a simplified mechanism of collagen cross-linking induced by the hydrogen bonding ability of TA is schematically depicted. At the same time, based on the peculiar structure with a large number of aromatic rings, TA exhibits a high hydrophobicity. Thus, there are experimental data that generally indicate a two-step process in collagen cross-linking by tannins: firstly, the tannin is adsorbed onto collagen fibres hydrophobically and, eventually, combines with collagen fibres by multi-hydrogen bonding [68]. Anyway, the overall effect of TA action onto collagen at either molecular or intermolecular level not only preserves the native structure of collagen, but also enhances it. Even though a general interaction mechanism that implies collagen, TA and CHDG is not an easy task at all, it is plausible that CHDG as a dication in aqueous environment (Fig. 9c) mainly interacts with the neighbouring components through both ion-dipole and hydrogen bond attractive forces, on one hand, and hydrophobic forces due to its hexamethylene bridge, on the other hand. As experimentally observed, all these types of forces occur without any detrimental effect on the native structure of collagen.
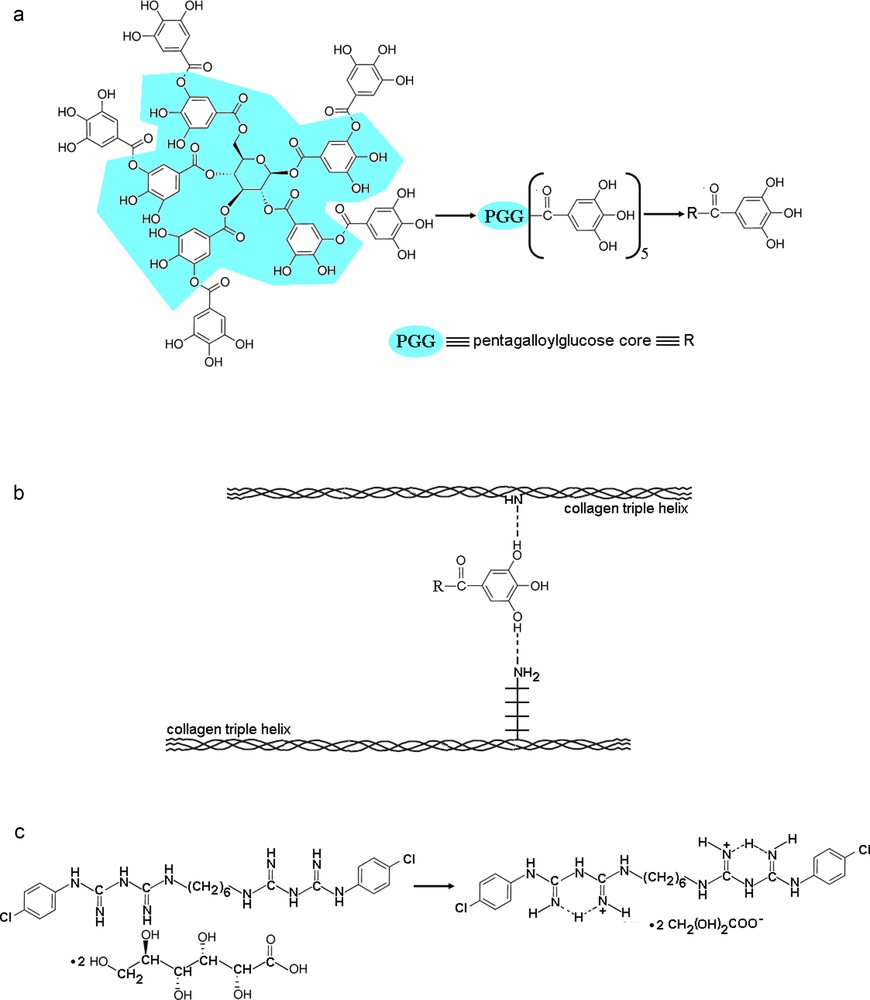
(Colour online). Overall structural characteristics of the components in the studied collagen-based hydrogels: (a) revealing the galloyl residues mostly implied in hydrogen bonding of TA; (b) a possible cross-linking mechanism involving two hydroxyl groups of TA and a =NH peptidic group and –NH2 side group belonging to two different collagen molecules; (c) particular cationic form of chlorhexidine in aqueous medium.
On the basis of structural and rheological properties, we believe that such collagen-based hydrogels are suitable to be applied topically and to be transformed into porous matrices or films.
4 Conclusions
FT–IR spectra of collagen hydrogels containing TA and CHDG showed that the used combinations of the two components did not alter the triple helix conformations of collagen.
At maximum amount of CHDG, its interaction with TA induced some changes in FT–IR spectra: splitting of amide II band and arising of a new band at 1541 cm−1 assigned to hydrogen bonding between OH phenolic groups of TA and amine groups of CHDG, on one hand, and a shoulder on the CH2 wagging band at 1470 cm−1 which was assigned to the interaction between TA and hexamethylene groups of CHDG by hydrophobic forces, that increased with TA concentration.
UV–CD spectra of the hydrogels also demonstrated the native triple helical conformation of the collagen. At the same time, the flattening of the peaks was due to the high concentration of collagen and incipient phase separation which produces the turbidity of hydrogels.
The hydrogels exhibited an overall pseudoplastic behaviour. However, all the hydrogels were easily destroyed under the action of shear rates higher than about 20 s−1 (in rotational viscometry tests), but displayed a prevalence of elasticity against viscous part in their viscoelastic properties.
The collagen-based systems were viscous enough to remain on the wound and quite elastic to be used on the regions in which the movement can otherwise produce the breaking of the applied layer of hydrogel. Therefore, the studied hydrogels based on non-denatured collagen could qualify as wound dressings, especially in the case of skin injuries.