1 Introduction
Volatile organic compounds (VOCs) and nitrogen oxides (NOx) arising from industrial gases are dangerous pollutants and have a detrimental effect on the environment and on human health. One of the most prominent results of VOCs and NOx emission is the formation of ground-level ozone, following the reaction between NOx and VOCs in the presence of sunlight. Excessive O3 in the air can cause breathing problems, trigger asthma, reduce lung function and cause lung diseases [1]. To overcome the problem, catalytic devices appear as an efficient technology for complete oxidation of VOCs or/and NOx reduction. Copper and iron species have been reported to be active in the selective oxidation of propene and/or in the reduction of NOx [2–4]. Moreover, their excellent low-temperature activity and cost effectiveness makes them a good substitute for noble metal catalysts. However, the most popular copper based catalyst, Cu-ZSM-5, discovered by Iwamoto et al. in 1993 [5], was believed to be the most promising catalyst for NOx decomposition, thus it did not appear resistant to H2O and SO2 present in flue gases from coal combustion [4].
In the past decade, mixed oxides derived from layered double hydroxides (LDHs) known as hydrotalcite-like compounds (HTLCs) have attracted much attention due to their large surface areas, good thermal stability, high dispersion, and basic character [6]. Among many applications, they are known to be powerful for hydrocarbons oxidation [7–9] and/or NOx reduction with hydrocarbons [2] or ammonia [10].
In this work, a series of CuxMg4–xAlFe catalysts derived from hydrotalcite-like compounds was synthesized, then characterized by various techniques. Subsequently, their performance was investigated in propene oxidation and in the simultaneous elimination of propene and NO. Previous studies [2,11] showed that mixed oxides derived from LDHs are potential catalysts for HC-SCR, other ones [3,4] demonstrated that copper and iron are very active species in this process. However, this work is the first to show the roles of copper and iron species coexisting in a single solid via hydrotalcite route in the HC-SCR.
2 Experimental
Mg(Cu)–AlFe hydrotalcite-like samples with a molar ratio equal to 2 were synthetized by a co-precipitation method. An aqueous solution containing appropriate amounts of Mg(NO3)2·6 H2O (FLUKA, 99%), Cu(NO3)2·6 H2O (PANREAC, 98%), Al(NO3)3·9 H2O (ACROS, 99%) and Fe(NO3)3·9 H2O (ACROS, 99%), was added dropwise into a vigorously stirred deionized water solution. During synthesis, temperature and pH were maintained constant at respectively 60 °C and 8 by dropwise addition of a solution of Na2CO3 1 M and NaOH 2 M. The obtained solution was stirred at 60 °C for a further hour, and then placed in the oven for 18 h. The precipitate was then filtered, washed several times with hot deionized water (55 °C) and dried at 60 °C for 48 h. The samples were denoted CuxMg4–xAlFeHT (0 < x < 4). The solids were then calcined at 500 °C under a flow of air (2 L·h−1).
X-ray diffraction experiments were performed at room temperature using a Bruker D8 Advance diffractometer equipped with a copper anode emitting a Kα radiation (λ = 1.5406 Å) and a LynxEye detector. A step size of 0.02° and a count time of 4 s per step were used for data collection. The crystalline phases were identified by comparing the diffractograms with the database of the Joint Committee on Powder Diffraction Standards (JCPDS) established by the International Center for Diffraction Data (ICDD).
The specific surface area of the dried and the calcined samples was determined by low-temperature nitrogen adsorption (−196 °C) using the Brunauer–Emmett–Teller (BET) method, with a thermo-Electron QSurf M1 sorptometer, while the Barett–Joyner–Halenda (BJH) pore diameters were determined by Sorptomatic 1990 sorption analyzer. Prior to the measurements, each sample was degassed at 400 °C for 3 h.
Scanning electron microscopy analysis was performed on a Mira4 Tescan instrument equipped with an energy dispersive X-ray spectrometer (SEM-EDX). Samples were adhered on aluminum stubs covered with 12-mm carbon adhesive tabs and then coated by a thin film of gold. Typical working parameters were an accelerating voltage of 20 kV, and a beam current of 60 μA.
The reducibility of the calcined samples was studied by H2 temperature-programmed reduction (TPR). H2-TPR experiments were carried out in an Altamira AMI 200 apparatus. Prior to the experiments, samples (10 mg) were activated under argon at 150 °C for 1 h. The samples were then heated from ambient temperature to 900 °C under a H2 flow (5 vol.% in argon-30 mL min−1) at a heating rate of 5 °C min−1.
In each test, a flow of the reaction mixture (6000 ppm propene + air for propene test and 6000 ppm propene + 1000 ppm NO + air for propene–NO test) passes through 100 mg of a catalyst placed in a fixed-bed reactor. The reaction products (NO, CO and CO2) were analyzed with Xentra 4900C analyzer (Servomex). The measurement of NO2 is done indirectly using a NO2 to NO converter BÜNOx (Bühler Technologies). Hence, the NOx quantity is obtained (NOx = NO2 + NO). Propene oxidation and N2O formation were checked using a Varian CP-4900 microGC.
3 Results and discussion
The XRD patterns of the CuxMg4–xAlFe precursors, presented in Fig. 1a, are characteristic of the hydrotalcite-like structure without any reflection of the other possible phases. Except for the sample where all the magnesium is replaced with copper, the presence of the malachite phase (Cu2(OH)2CO3) is also revealed due to the Jahn–Teller effect in the Cu2+. Indeed, Carpentier et al. [12] reported that when all the magnesium in the precursor is replaced by copper, the malachite phase is preferentially formed. The formation of layered materials is evidenced by the sharp, symmetric, strong lines at low 2θ values and weaker, less symmetric lines at high 2θ values [2,12]. The diffraction peaks at 2θ ≈ 11°, 23°, 34°, 39°, 46°, 60°, 61° can be attributed to the (003), (006), (012), (015), (018), (110) and (113) crystal planes for HT phase, respectively [13]. Fig. 1b shows the X-ray diffractograms recorded for the CuxMg4–xAlFe 500 solids. After calcination, the hydrotalcite and the malachite phases are transformed into a mixture of oxides and spinels of type: MgO (JCPDS 75-1525), CuO (JCPDS 80-0076), -Fe2O3 (JCPDS 04-0755) or/and Fe3O4 (JCPDS 65-3107), MgFe2O4 (JCPDS 36-0398) and CuFe2O4 (JCPDS 77-0010). However, with further increasing of Cu content, the diffraction peaks of CuO and CuFe2O4 get sharper and replace those of MgO and MgFe2O4. Similar diffractograms were obtained by Jablonska et al. [10] after calcination of Cu–Mg–Fe HTLCs at 600 ˚C.
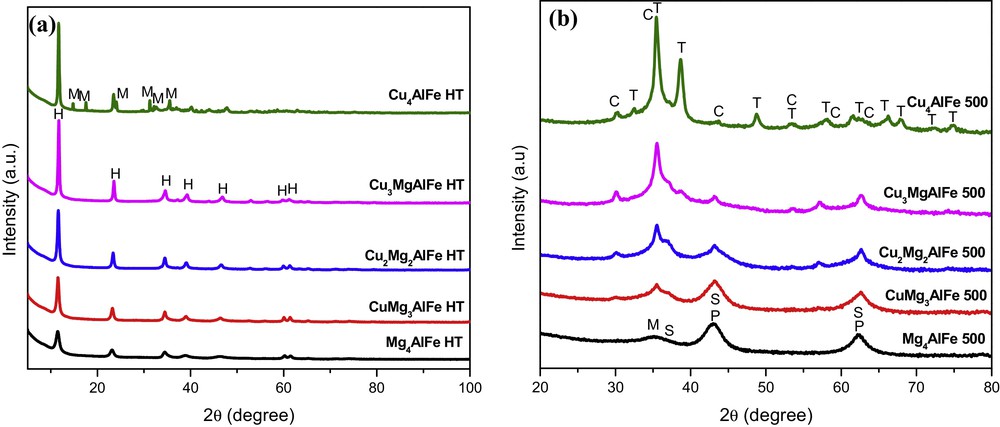
(Color online.) X-ray diffraction (XRD) patterns of: a: CuxMg4–xAlFe HT hydrotalcite-like compounds; b: CuxMg4–xAlFe 500-mixed oxide catalysts. H: hydrotalcite; M: malachite; P: MgO (periclase); T: CuO (tenorite); M: Fe3O4 (magnetite) and/or -Fe2O3 (maghemite); S: MgFe2O4 (magnesium iron oxide); C: CuFe2O4 (cuprospinel).
The physical properties of the as-synthesized and calcined hydrotalcite-like compounds were evaluated by BET and by N2 adsorption/desorption measurements, and the results are listed in Table 1. As can be seen, the surface area of the samples given by the BET technique decreases after calcination. The areas of the calcined hydrotalcites ranged from 49 to 80 m2·g−1; however SBET decreases gradually with increasing the copper content. This result can be related to the higher crystallization of the samples with higher copper species, as it was revealed by XRD. The results from N2 adsorption/desorption experiments showed that all the samples exhibited type-IV isotherms, which are characteristic of mesoporous materials. Average pore diameters, as well as pore volumes, are presented in Table 1.
Physical properties of the various samples.
Samples | BET surface area of dried samples (m2·g−1) | BET surface area of calcined samples at 500 °C (m2·g−1) | Pore volume (cm3·g−1) | Average pore diameter (nm) |
Mg4AlFe | 117 | 80 | 0.62 | 3.37 |
CuMg3AlFe | 95 | 75 | 0.49 | 1.98 |
Cu2Mg2AlFe | 78 | 70 | 0.26 | 2.12 |
Cu3MgAlFe | 82 | 51 | 0.19 | 1.96 |
Cu4AlFe | 76 | 49 | 0.15 | 1.94 |
The sharp decrease in the pore volume is related to the sintering and the formation of spinel phases when copper is substituted for magnesium. According to Valente et al. [14], the porosity of the calcined samples is due to two different processes: small intraparticle porosity is generated by a “cratering” process, and the interparticle pores are created by the irregular stacking of the plate-like particles.
The morphology of the hydrotalcite-like compounds is revealed by SEM and is shown in Fig. 2. SEM images show highly crystalline particles. All the samples, with the exception of Cu4AlFe HT, showed a flower-like hierarchical morphology typical of LDHs. Similar shapes were obtained by Gennequin et al. [6] for Mg–Al hydrotalcite. However, Cu4AlFe HT showed a mixture of crystalline and irregular nanoflakes structures, in accordance with the XRD results, which revealed the presence of both hydrotalcite and malachite phases.
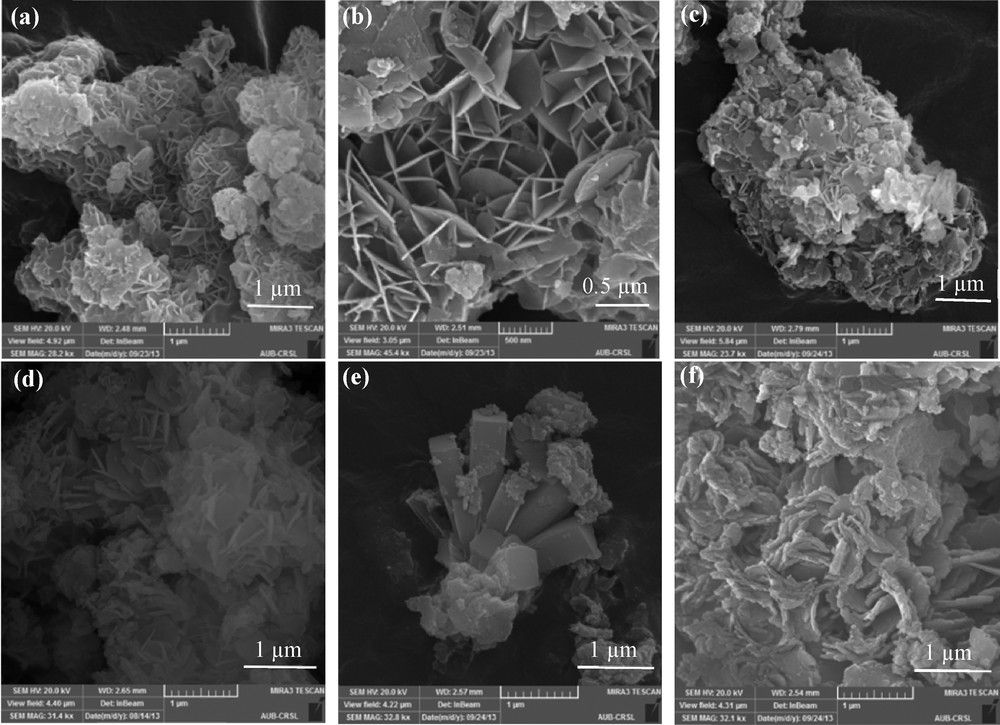
Scanning electron microscopy (SEM) micrographs of the CuxMg4–xAlFe HT samples: a: Mg4AlFe HT; b: CuMg3AlFe HT; c: Cu2Mg2AlFe HT; d: Cu3MgAlFe HT; e and f: Cu4AlFe HT.
The redox properties of the samples were investigated by temperature-programmed reduction, with the H2-TPR profiles shown in Fig. 3. The curves of the catalysts display two reduction regions: the reduction of the copper oxide species present in the forms of CuO and CuFe2O4 occurs in the low-temperature range (T < 350 °C), while the reduction of iron oxide phases present in the forms of -Fe2O3 or/and Fe3O4 appears at higher temperatures (T > 350 °C) [10]. The reduction peaks due to copper and iron species were deconvolved, and the curve-fitting results are summarized in Table 2. The peaks below 200 °C (peak α and peak β) correspond to the stepwise reduction of highly dispersed CuO species (isolated copper ions and small clusters) i.e., Cu2+ → Cu+ and Cu+ → Cu0 [2]. Moreover, the peaks at 217 °C, 222 °C and 263 °C are attributed to more aggregated copper oxide phases such as bulky CuO [3] and the reduction of CuFe2O3 into metallic copper and Fe2O3 (peaks δ and ). On the other hand, the peaks at higher temperatures are ascribed to the reduction of Fe2O3 into Fe3O4 and of Fe3O4 into 3 FeO (peak φ) [15]. It can be noted that H2 consumption increases with enhancing the copper content.
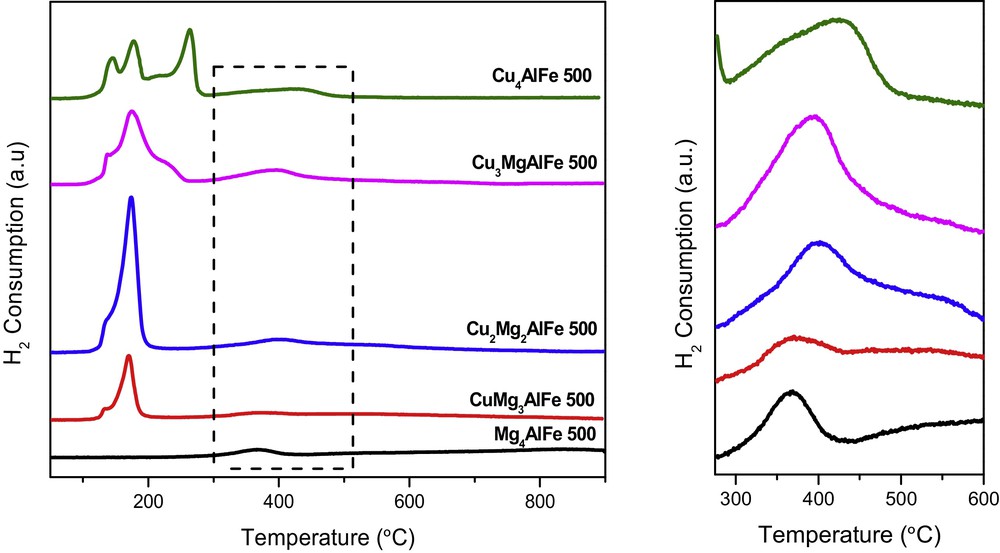
(Color online.) H2-temperature-programmed reduction (TPR) profiles of the calcined samples.
The temperature and the H2 consumption corresponding to the peaks in H2-TPR.
Samples | Temperature (°C) [H2 consumption (μmol·g−1)] | Total H2 consumption (μmol·g−1) | ||||
α | β | δ | φ | |||
Mg4AlFe 500 | 368 (729.9) | 729.9 | ||||
CuMg3AlFe 500 | 134 (538.3) | 170 (2453.6) | 371 (1970.6) | 4962.5 | ||
Cu2Mg2AlFe 500 | 133 (757.8) | 174 (8326.6) | 401 (1290.6) | 10375 | ||
Cu3MgAlFe 500 | 138 (698.4) | 175 (6892.7) | 222 (899.1) | 397 (2449.5) | 10939.7 | |
Cu4AlFe 500 | 145 (1901.6) | 177 (3043.2) | 217 (2128.8) | 263 (3576.9) | 426 (1753.1) | 12403.6 |
The catalytic activity of CuxMg4–xAlFe 500 was studied in the oxidation of propene.
Fig. 4a represents the conversion of propene versus temperature for the different supports in the absence of NO. The light-off curves (temperatures in which 50% of the propene is converted) and the intrinsic activity are shown in Table 3. The temperature used for the calculation of the intrinsic activity corresponds to a propene conversion of 20% of the catalyst that exhibited the best activity. It was observed that the introduction of copper enhanced the catalytic performance, which attests to the fact that copper and iron oxide species as well as the spinel phase CuFe2O4 play an important role in this process. Compared to the other supports, Mg4AlFe showed the lowest catalytic activity. However, the better activity of the samples with high copper content is due to their higher reducibility, which promotes a redox cycle favorable to the oxidation of propene in the presence of air, as shown in the TPR profiles.
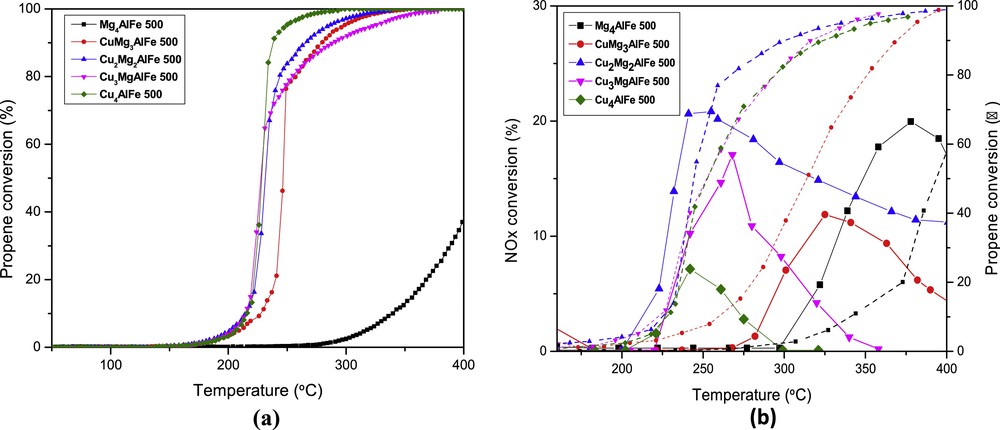
(Color online.) Catalytic performance of the catalysts CuxMg4–xAlFe 500 as a function of temperature: a: propene conversion (%) in the absence of NO; b: NOx conversion (—) and propene conversion (- - -).
Light-off temperatures and intrinsic activities of propene conversion in the absence and in the presence of NO over the Mg(Cu)–AlFe mixed oxide catalysts.
Catalysts | Mg4AlFe 500 | CuMg3AlFe 500 | Cu2Mg2AlFe 500 | Cu3MgAlFe 500 | Cu4AlFe 500 |
T50 (°C) without NO | 419 | 247 | 231 | 227 | 228 |
Intrinsic activity (mol·s−1·m−2) | 4.9 10−9 | 2.37 10−7 | 6.41 10−7 | 1.49 10−6 | 1.13 10−6 |
T50 (°C) with NO | 395 | 315 | 245 | 250 | 253 |
Intrinsic activity (mol·s−1·m−2) | 1.2 10−8 | 2.57 10−7 | 8.98 10−7 | 1.96 10−6 | 1.16 10−6 |
Concerning propene oxidation, it is to be noted that when the conversion is complete, H2O and CO2 are the only products observed.
Calcined hydrotalcites were then tested as catalysts in the simultaneous elimination of propene and NO. Fig. 4b shows the conversion of NOx (—) and propene conversion (- - -) as a function of the reaction temperature. With increasing temperature, NO conversion enhanced until a maximum was reached. After that, it declined, because propene is nearly completely oxidized. When Cu loading was increased (from x = 1 to x = 2), the maximum of NO conversion increased and T50 decreased. After reaching an optimum value for copper content (x = 2), the maximum NO conversion started to decrease and T50 increased (Table 3). This can be explained by the fact that, when the copper quantity in the sample increase, this leads to the aggregation of the surface CuO, thus blocking the active sites for propene oxidation and NO reduction. Furthermore, during the simultaneous elimination of C3H6 and NO, the competition on the metallic sites makes NO reduction more difficult. Hence, the best activity is obtained for Cu2Mg2AlFe 500, where the maximum of NO conversion is 21% at 255 °C and T50 is 245 °C. This catalyst exhibited the best activity because it contains the highest amount of surface copper species. A study by Yuan et al. [2] showed similar results in the catalytic reduction of NO by C3H6 over CuxTi1 mixed oxides derived from hydrotalcite-like compounds catalysts. They showed that surface Cu species are the active sites, while bulk Cu species could provide the adsorption sites for nitrogen species. Moreover, Worch et al. [16] studied the SCR of NO with propene over transition metal oxides (Fe-, Cu-, V-, and Ce-) supported on Al2O3, phosphated Al2O3, or SAPO-11. The results shown in Fig. 4b presented a relatively better NO conversion and propene oxidation rates at lower temperatures compared to the study of Worch et al. Therefore, it is to be pointed out that the presence of both copper and iron species in a single solid has resulted in lower temperatures of complete propene conversion and in a better NO conversion. Other studies [10,15] showed that mixed metal oxides of Cu, Mg, Al and Fe derived from hydrotalcite-like compounds, tested in the selective catalytic oxidation of ammonia to dinitrogen, are active in the process of NO reduction with ammonia to N2 and N2O. The activity of the catalysts depended on their chemical composition, mainly on the presence of copper oxide phases, and on their redox properties.
It should be noted that N2, CO2, and H2O were the main products of the reactions; however, traces of CO and N2O in the reaction products were also found.
4 Conclusion
Mg(Cu)–AlFe hydrotalcite-like compounds with different copper contents were successfully synthesized. Their thermal decomposition at 500 °C leads to the formation of metal oxides and spinels that play a major role in propene oxidation and NOx reduction. In propene oxidation, the increase in copper loading enhanced the catalytic performance of the supports. This was explained by a higher reducibility of those solids and to the presence of oxide phases such as CuO and CuFe2O4. As for the simultaneous elimination of C3H6 and NO, the Cu2Mg2AlFe 500 presented the highest activity; this is mainly due to the presence of well-dispersed surface copper and iron species. Hence, increasing copper concentrations leads to agglomerated copper species, thus reducing the activity of the catalysts. The coexistence of copper and iron in a single material leads to an interesting activity for NOx reduction by propene, but the Cu/Fe ratio should still be adjusted to increase the catalytic properties.
Acknowledgements
This work has been supported by the Lebanese National Council for Scientific Research, the Université du Littoral Côte d’Opale, the Institut de recherche en environnement industriel (IRENI), and the European Union (Interreg 4 France–Wallonie–Flandre project, REDUGAZ).