1 Introduction
Magnetite [1] is a well-known material that possesses a highly active surface for the immobilization and adsorption of catalytic fragments, including organocatalysts, enzymes, and metal catalysts (such as gold, palladium, platinum, copper, nickel, cobalt, and iridium), which results in the formation of remarkably sustainable catalysts. It has been used as a versatile catalyst support in a wide variety of reactions, including hydrogenation, reduction, oxidation, cycloaddition, asymmetric synthesis, and the Suzuki, Heck, Sonogashira, and Hiyama [2–4]. Recently, the application of copper-based nanoparticles as a catalyst has generated much interest. The possible modification of the chemical and physical properties of the nanoparticles has been largely responsible for the rapid growth of interest in these nanomaterials and their applications in catalysis [5].
Propargylamines are prominent components strikethrough as precursors for the synthesis of various nitrogen-containing compounds (such as allylamines, pyrrolidines, oxazoles, and pyrroles) or as intermediates in the preparation of a diverse range of natural products, biologically active compounds, and pharmaceuticals (Scheme 1). In addition, some propargylamines have been found to possess interesting biological properties (e.g., as inhibitors) and have even been tested as targets for multifunctional drugs in the treatment of Alzheimer's and Parkinson's diseases [6–9].
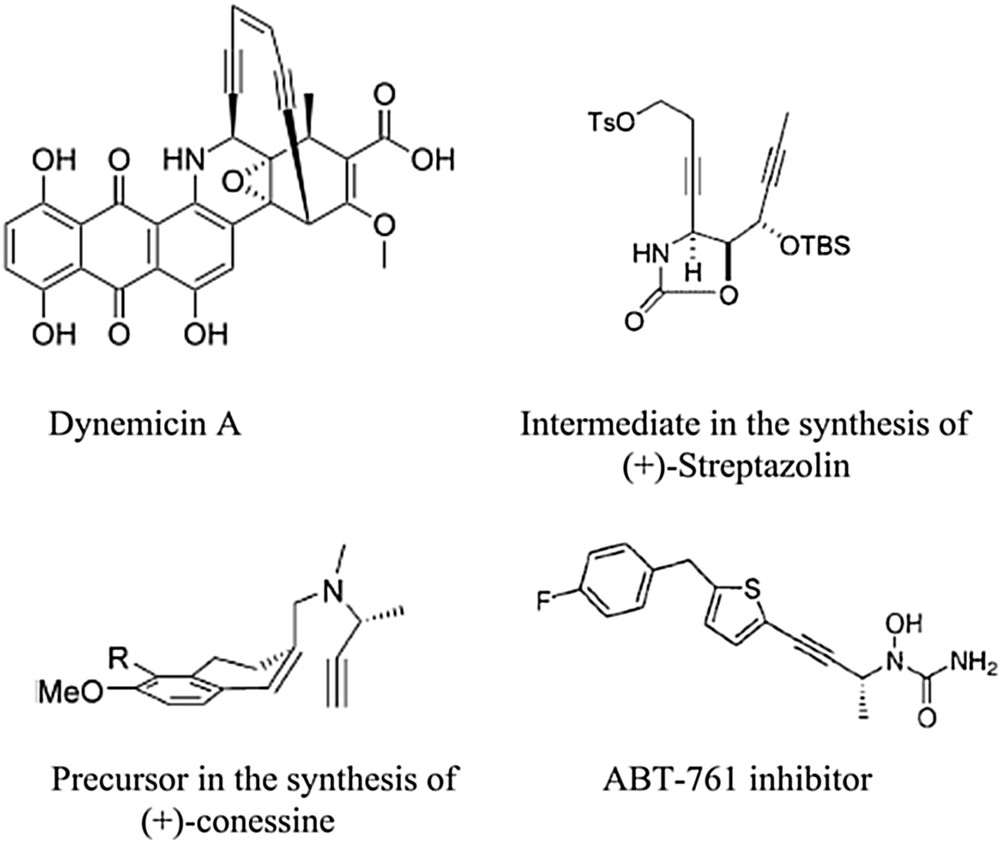
Selected compounds with propargylamine skeleton [10–13].
The catalytic coupling of the aldehyde–alkyne–amine (A3 coupling), the addition of alkyne nucleophiles to CN electrophiles, has shown great utility in the simple and rapid preparation of propargylamines in broadly tolerant and highly tunable reaction conditions [14]. In this regard, several catalytic systems using transition metal catalysts have been developed [15–18]. A review of the literature has indicated that copper catalysts have played a noteworthy role in the development of methods for propargylamine synthesis [5,8,19–28]. In this study, we report the synthesis and characterization of copper-based magnetic nanoparticles (MNPs) and document their catalytic application in propargylamine synthesis.
2 Experimental section
2.1 General
All chemicals used in this study were purchased from commercial sources and used without further purification. The reaction was monitored by thin-layer chromatography (TLC) on aluminum-backed silica gel 60 F254 plates (0.2 mm thickness, Merck). Atomic absorption measurements were performed using a Shimadzu AA-6300 spectrometer. Scanning electron microscopy (SEM) and energy dispersive X-ray (EDAX) analyses were carried out using a Philips XL30 instrument for the elemental analysis of the nanoparticles. The transmission electron microscopy (TEM) image was recorded using a Zeiss-EM10C at 80 kV. Magnetism analysis was performed using a vibrating sample magnetometer (4 in., Daghigh Meghnatis Kashan Company, Kashan, Iran) at room temperature. The Fourier transform infrared (FT-IR) spectra were acquired on a BOMEM MB-Series 1998 FT-IR spectrometer using KBr pellets.
2.2 Synthesis of Cu–imine ligand@SiO2@Fe3O4
To introduce a linker, SiO2@Fe3O4 (2 g) was dispersed in dry toluene (10 mL) and sonicated for 15 min in an ultrasonic bath. After this, (3-aminopropyl) triethoxysilane (2 mL) was added to the mixture, which was then refluxed for 72 h under nitrogen. The resultant particles were separated magnetically, washed thoroughly with ethanol, and dried under reduced pressure to obtain Am-SiO2@Fe3O4. The surface was subsequently modified by adding Am-SiO2@Fe3O4 (1 g) to a solution of 2-hydroxybenzaldehyde (1 mmol, 0.122 g) in ethanol (10 mL), and the mixture was then stirred under reflux conditions for 12 h. The resultant MNPs were removed using an external magnet, washed repeatedly with ethanol, and dried to generate imine ligand@Am-SiO2@Fe3O4. Finally, to synthesize copper(II) chelate-bonded Fe3O4 nanoparticles, a mixture of Azo ligand@Am-SiO2@Fe3O4 (2.0 g) in ethanol (10 mL) was sonicated for 15 min. Then, copper(II) chloride (1 mmol, 0.134 g) was added, and the resulting mixture was refluxed for 24 h. After the particles were magnetically removed, the nanocomposites were washed with water and dried under vacuum.
2.3 General procedure for the synthesis of propargylamines
In a test tube, a mixture of aldehyde (1 mmol), phenylacetylene (1.3 mmol), and piperidine or morpholine (1.3 mmol) was reacted in the presence of copper(II)–imine ligand@SiO2@Fe3O4 (0.1 g) under solvent-free conditions at 80 °C. After the reaction was complete (as monitored by TLC), the reaction mixture was allowed to cool to room temperature and diluted with DCM (10 mL). The MNPs were then separated magnetically, and the organic phase was washed with water, dried over Na2SO4, and concentrated under reduced pressure. The final product was then subjected to column chromatography (1:4 v/v ethyl acetate/hexane) for further purification.
3 Results and discussions
Our strategy for immobilizing a copper(II)–imine complex onto silica-coated magnetite was based on our previous work (Scheme 2) [29–31]. The synthetic method commenced with the surface modification of SiO2@Fe3O4 with (3-aminopropyl) triethoxysilane, which was subsequently reacted with salicylaldehyde. We assumed that these treatments in addition to forming an active site of imine and hydroxyl groups for chelate with copper enhance the lipophilicity of the nanoparticles.

Synthesis of copper(II) chelate-bonded magnetite nanoparticles.
The resulting MNPs were analyzed and characterized by analytical techniques including SEM, EDAX, FT-IR, thermogravimetric and derivative thermogravimetric (TG-DTG) analyses, and vibrating sample magnetometry.
A particle size with about ∼41–76 nm diameter with a nearly spherical shape was observed in the SEM and TEM images of the CuPs. The presence of the main elements (iron, oxygen, silicon, nitrogen, carbon, copper, and chlorine) was confirmed by EDAX analysis (Fig. 1). In addition, the copper content was determined to be ∼2.5 wt % by atomic adsorption spectroscopy.

SEM/EDAX elemental analysis and TEM image of Cu–imine ligand@SiO2@Fe3O4.
In the FT-IR spectrum of the copper nanoparticles various peaks in the range of 400–4000 cm−1 corresponded to the magnetite and outer silica layers and to the linker/ligand parts (Fig. 2). The band at ∼586 cm−1 belonged to FeO stretching vibration, an intense band at ∼1105 cm−1 was because of SiOSi antisymmetric stretching vibrations, and the presence of a broad band at ∼3350 cm−1 was attributed to the SiOH group. Also, bands at ∼2850–2926 cm−1, weak bands at ∼1460–1487 cm−1, and a shoulder band at ∼1617 cm−1 were associated with CH, CC, and CN stretching vibration, respectively. In addition, the band at ∼1623 cm−1 was attributed to the water molecules that were adsorbed on the surface [32].
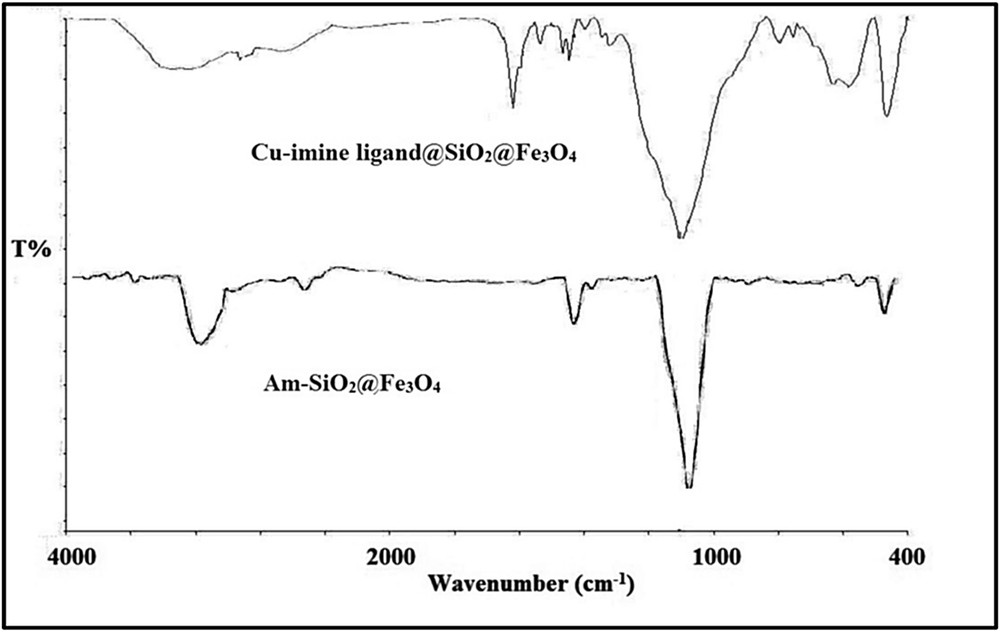
FT-IR spectra of Am-SiO2@Fe3O4 and Cu–imine ligand@SiO2@Fe3O4.
The thermal stability of the Cu(II)–imine ligand@SiO2@Fe3O4 was examined by TGA-DTG under nitrogen atmosphere (Fig. 3). The TGA-DTG data indicate mass loss corresponding to the removal of physically adsorbed water up to ∼150 °C and another one between ∼260 and 400 °C that can be attributed to the rapid thermal decomposition of the linker and ligand moieties.

TG-DTG analysis for Cu–imine ligand@SiO2@Fe3O4.
A vibrating sample magnetometer was used to measure the magnetic property of the Cu NPs. It can be seen that the magnetization curves appeared s-shaped over the applied magnetic field. The samples exhibited typical superparamagnetic behavior by showing zero coercivity. This means that they were related to an external magnetic field but retained no residual magnetism once the external magnetic field had been removed at room temperature [33]. In comparison with the neat Fe3O4, the saturation magnetization value of copper(II) chelate-bonded Fe3O4 decreased from ∼60 to ∼24 emu/g, which can be attributed to the coating of nonmagnetic materials on the magnetite particles (Fig. 4).
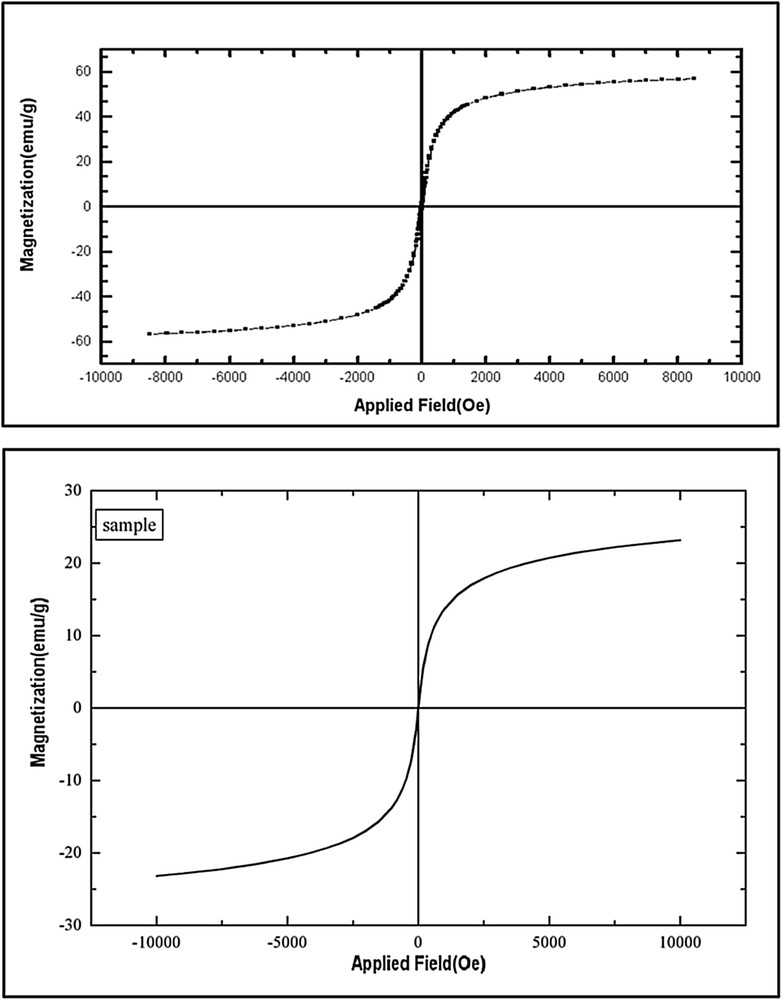
The magnetic curves of Fe3O4 (upper) and Cu–imine ligand@SiO2@Fe3O4 (lower).
It has been demonstrated that the use of solvent-free conditions in heterocyclic synthesis generally leads to similar or higher yields compared to the same reaction performed in the presence of molecular solvents; the reaction time is also reduced [34]. Accordingly, we performed a screening of the reaction conditions from the viewpoint of temperature, amount of reactant, and catalyst at the outset of this work. As expected, the treatment of benzaldehyde, piperidine, and phenylacetylene without catalyst showed no reaction even at a high temperature (100 °C) over the course of a lengthy reaction time (24 h). In addition, yields decrease dramatically at lower reaction temperatures. The scope of the reaction was then explored under the optimized conditions using a variety of aromatic aldehyde, phenylacetylene, piperidine and/or morpholine, and MNPs as catalysts (Table 1).
Scope of the synthesis of propargylamines catalyzed by Cu(II)–imine ligand@SiO2@Fe3O4.
One of the most disadvantages of the use of immobilized homogeneous catalysts is that the valuable metal is continuously leached and carried away with the products [35]. To check this issue, a mixture of Cu NPs (1.0 g) in EtOAc (10 mL) was refluxed for 12 h, the nanoparticles were then separated using an external magnet, and the residue was analyzed by atomic adsorption spectroscopy. It was found that only a negligible amount of copper (<1 ppm) was leaching.
The synthesis of 4g was selected as the model for reusability test. It was performed for five consecutive runs. On each run, the reaction was allowed to proceed to complete conversion. The catalyst was carefully separated and washed with EtOAc after the completion of reaction. The results showed that the reaction time increased with a gentle slope from 8:15′ to 8:45′ h (Fig. 5).

Reusability studies.
In conclusion, we have developed an efficient method of using a multicomponent reaction to synthesize propargylamine derivatives with good to high yields. The reaction was catalyzed by a novel magnetite-supported copper nanoparticle under solvent-free conditions. The catalyst was recovered from the reaction medium using an external magnet and reused for five runs with acceptable catalytic activity.
Acknowledgments
We gratefully acknowledge the financial support of the Mahshahr Branch, Islamic Azad University. We are also grateful to Prof. Paula S. Branco of the New University of Lisbon for her helpful comments.