1 Introduction
Carbohydrates are important compounds in nature both by their particular structures and by multivalent interactions [1]. Specifically, the synthesis and study of new carbohydrate/metal complexes stable in aqueous media are important not only at a biological level [2,3] but also at an environmental level, see for instance their impact on the paper industry [4–6]. In particular, the search for new complexes of iron(III) with sugars as ligands is of interest given the vital importance of this metal [7]. On the other hand, the quantitative characterization of sugar/metal complexes containing only oxygen donor atoms (hydroxyl, aldehyde or ketone groups) proves difficult because sugars form unstable complexes with metallic centres due to the low electron densities on donor oxygen present in these organic molecules in neutral or acidic aqueous media [8]. However, at high pH and after the oxygen deprotonation of one hydroxy group of a carbohydrate, the formation of complexes can be expected and this prevents the hydrolysis of metal ions [9].
The stability of these carbohydrate/metal complexes is influenced by the fraction of isomers in solution, the hemiacetal ring size and the relative position of the hydroxyl groups, cis or trans, to each other [10]. It is also known that the effect of each of the factors mentioned previously is specific for each metallic centre [11–13].
Cyclodextrins (CDs) are cyclic oligomers of d-glucose, bonded by α–1-4 linkages. They have a toroidal structure similar to a truncated cone. Their interiors behave as a hydrophobic cavity, whereas the two, three and six hydroxyl groups of the glucose units are located at the edges of the truncated cone and define the hydrophilic zones of the oligomer [14–17].
The importance of metal/CDs complexes has been highlighted in a recent review where these complexes are proposed as the basis of novel materials [18]. Although β-CD (oligomer with seven glucose units) has no isomers and thus its use as an organic ligand would simplify structural problems, difficulties can be found in the isolation and characterization of these complexes; hence, the study of their reactivity and potential applications is relatively new [19–24].
Complexes between transition metals and different CDs have been reported [25–27] and some have been used in organic reactions carried out in aqueous solutions [28,29]. For example, the behaviour of Cu(II) complexes with β-CD [30], xylitol [31], different monosaccharides [32] and dextran, a carbohydrate polymer [33,34], has been studied in basic solution. In addition, CDs have recently been used in the preparation of cobalt–zirconia catalysts [35].
In previous studies [36], we have reported the stabilization of FeBr3 in a β-CD basic aqueous solution with a β-CD/FeBr3 concentration ratio greater than 3. That was explained by the existence of some type of complex between β-CD, or its anion, and iron(III) bromide. We report here studies of the interaction of different metal salts of the first transition-metal series and β-CD. The results found are interesting because different metallic cations, which usually precipitate in basic medium, could be kept in a solution when β-CD was added. This is particularly important because it could be possible to use transition metals in basic aqueous medium as catalysts in the homogeneous phase.
2 Experimental section
2.1 Equipment and chemicals
UV–vis spectra were performed using a Shimadzu UV-2101PC spectrophotometer. The experiments were carried out in a thermostatic bath at (25.0 ± 0.1) °C. The reagents were commercially available samples and used as received: β-CD (Roquette, Lestrem, France); NaOH (Merck); MnBr2 anhydrous, Mn(NO3)2 anhydrous, FeBr3 anhydrous, Fe(NO3)3·9H2O, CoCl2 anhydrous, Co(NO3)2·6H2O, NiBr2 anhydrous, NiCl2 anhydrous, Ni(NO3)2·6H2O, Co(NO3)2·6H2O, CuCl2·2H2O, Cu(NO3)2·2.5H2O, FeBr2 anhydrous (Aldrich); MnCl2 anhydrous, FeCl3 anhydrous, CoBr2·H2O 16.4%, CuBr2 anhydrous (Strem Chemical); ZnBr2 anhydrous (Alfa Aesar). The water used was purified in a Milli Q apparatus.
2.2 General procedure
The basic solutions used were 1 × 10−2 M NaOH (solution A).
The β-CD solutions (solution B) were made up using solution A and β-CD. This solution was used to dissolve salts to provide adequate concentrations for the studies.
The metal salts (≈2 × 10−5 mol) were dissolved in 10, 25 or 50 mL of basic solution (solution A or solution B as appropriate) and placed in the water bath at 25 °C in a graduated flask. To obtain the electronic spectrum, 1 mL of the solution under study was diluted to 10 mL with solution A or solution B as appropriate.
Experiments in imidazole buffer were made by dissolving the metal salt in the buffer directly or in the same buffer containing approximately 1 × 10−2 M β-CD.
Unless otherwise stated, spectroscopic studies were carried out for 24 h.
3 Results and discussion
We have studied the bromides, chlorides and nitrates of manganese(II), iron(III), cobalt(II) and nickel(II), and the bromide of iron(II) and zinc(II). The experiments were carried out in basic aqueous solution and basic solution containing different concentrations of β-CD. These salt solutions were studied by UV–vis spectrophotometry.
3.1 Manganese
Manganese(II) is a stable cation in neutral, acid [37] or slightly basic aqueous media; however, at pH values greater than 8.5, the hydroxide precipitates [38]. Furthermore, the oxidation of the hydroxide occurs readily under alkaline conditions due to the corresponding decrease in redox potential. When Mn(OH)2 is oxidized in air, it forms a brown hydrated oxide with stoichiometry MnO(OH) [39]. This mineral, called manganite [γ-MnO(OH)], contains a formal Mn3+ and magnetic measurement suggesting the presence of both Mn2+ and Mn4+ [39].
In this study, we used three manganese salts, manganese(II) bromide, chloride and nitrate. As expected, when MnBr2 was dissolved in NaOH solution, a dark brown precipitate was immediately formed, accompanied by a decrease in absorbance (Fig. 1S). MnCl2 had similar behaviour (Fig. 3S). On the other hand, the basic solution of Mn(NO3)2 was clear, yellowish and the precipitate was not observed for 24 h (Figs. 1 and 5S).
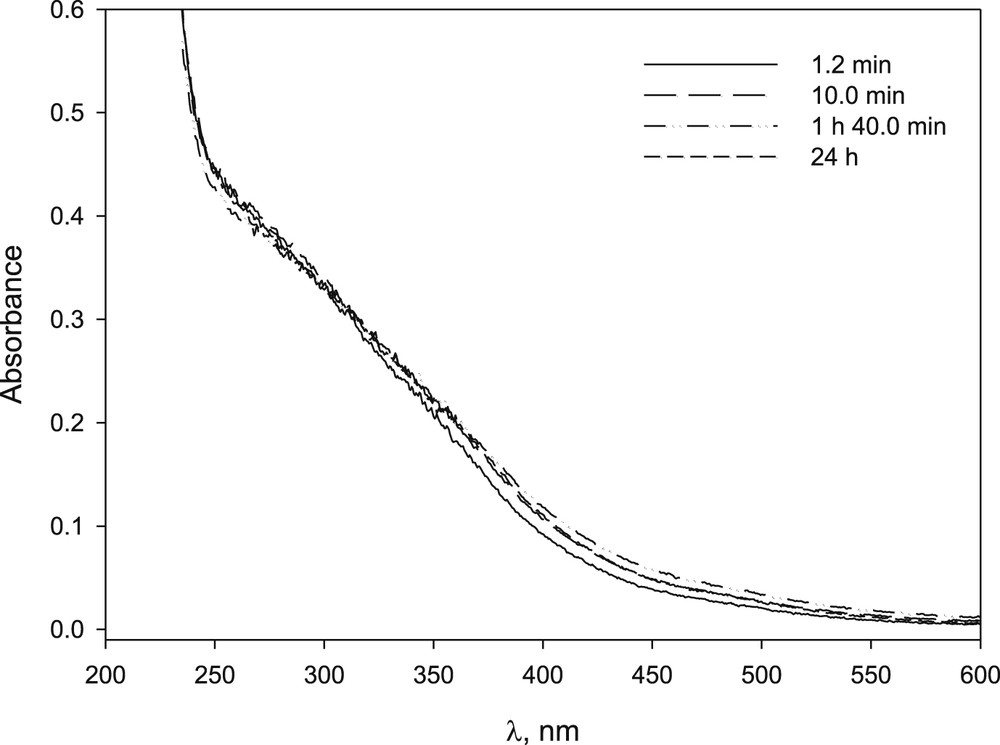
UV–vis spectrum of Mn(NO3)2 in NaOH solution at different time after preparation: [Mn(NO3)2] 3.4 × 10−4 M, [NaOH] 9.94 × 10−3 M.
When a basic solution of β-CD was used to dissolve the manganese salts, metal precipitation was avoided for a few hours (approximately 5 h); β-CD/MnX2 molar ratio was 1/1. In both cases, with MnBr2 or MnCl2, an increase in absorbance and a change in the shape of the UV–vis spectrum was observed; however, after 24 h, a precipitate of similar appearance to that observed in an aqueous basic solution was formed (see Figs. 2S and 4S). The immediate precipitation of the metal salt was prevented by the presence of β-CD. Probably a complex was formed between the metal salt and β-CD because an increase in absorbance was observed in the wavelength area corresponding to the ligand–metal charge transfer transitions.
3.2 Iron
Iron(III) forms salts with most anions and complexes with both inorganic and organic ligands. The greatest iron affinity is for ligands that coordinate through oxygen, such as phosphate ions, polyphosphates and polyols [39]. One of the most notable characteristics of iron(III) behaviour in aqueous solution is its tendency to hydrolyze [40]. In solutions with pH values greater than 2, colloidal species are formed and finally precipitated as a reddish-brown gelatinous mass, in the form of hydrated iron trioxide(III). Particularly, when iron(III) salts are dissolved in aqueous alkaline solution, they generally precipitate as basic salts.
Fe(III) species formed in alkaline aqueous medium have been extensively studied because of their importance both in physiological and in environmental conditions [41–43]. In addition, the behaviour of Fe(III) in aqueous solutions at high pH is of great interest because it is considered a contaminant in industrial processes where strong alkaline media are used [44–49].
The solid complex of β-CD–Fe, prepared according to Ref. [36], was dissolved in basic medium and its UV–vis spectrum was practically unchanged for 24 h, indicating that there was no precipitation (Fig. 2). Ferric bromide dissolved in basic media produced, over time, the appearance of precipitate and the loss of the typical yellow colour of these solutions with a significant change in UV–vis spectrum (Fig. 3). Yet, when FeBr3 was dissolved in a basic solution of β-CD, it did not precipitate after 24 h and the changes observed in spectrum UV–vis were slight even after 30 h (Fig. 4). Although the amount of FeBr2 used was not completely dissolved, the spectrum obtained did not change during the period studied (Fig. 6S).
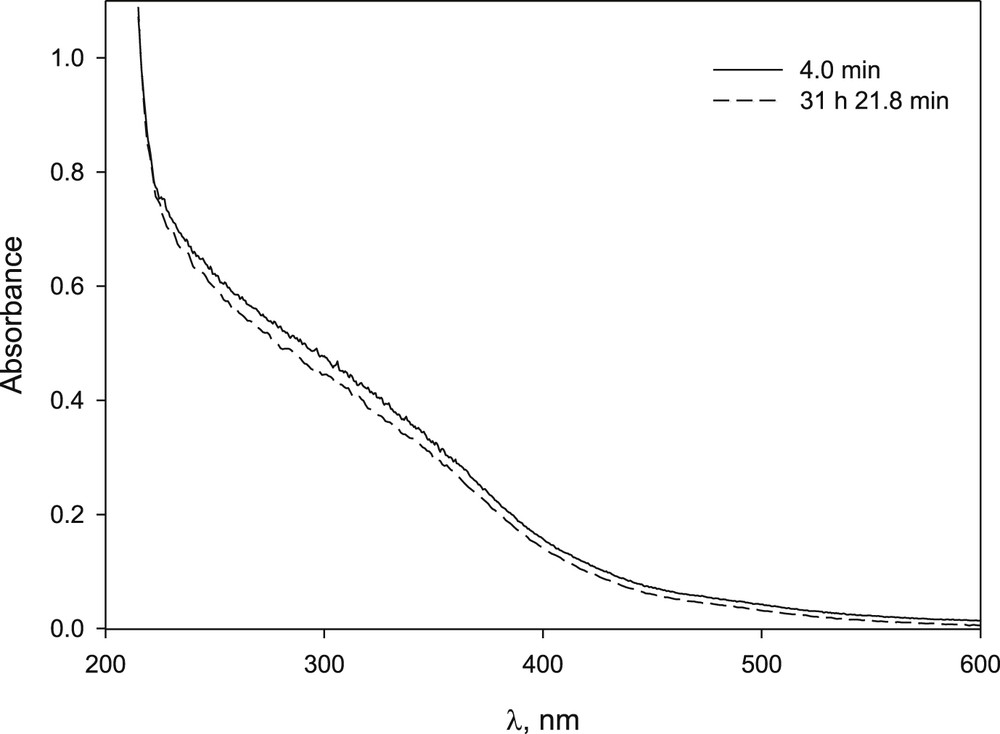
UV–vis spectrum of β-CD–Fe complex in NaOH solution at different time after preparation: [complex β-CD–Fe] 1.8 × 10−4 M, [NaOH] 1.0 × 10−2 M.
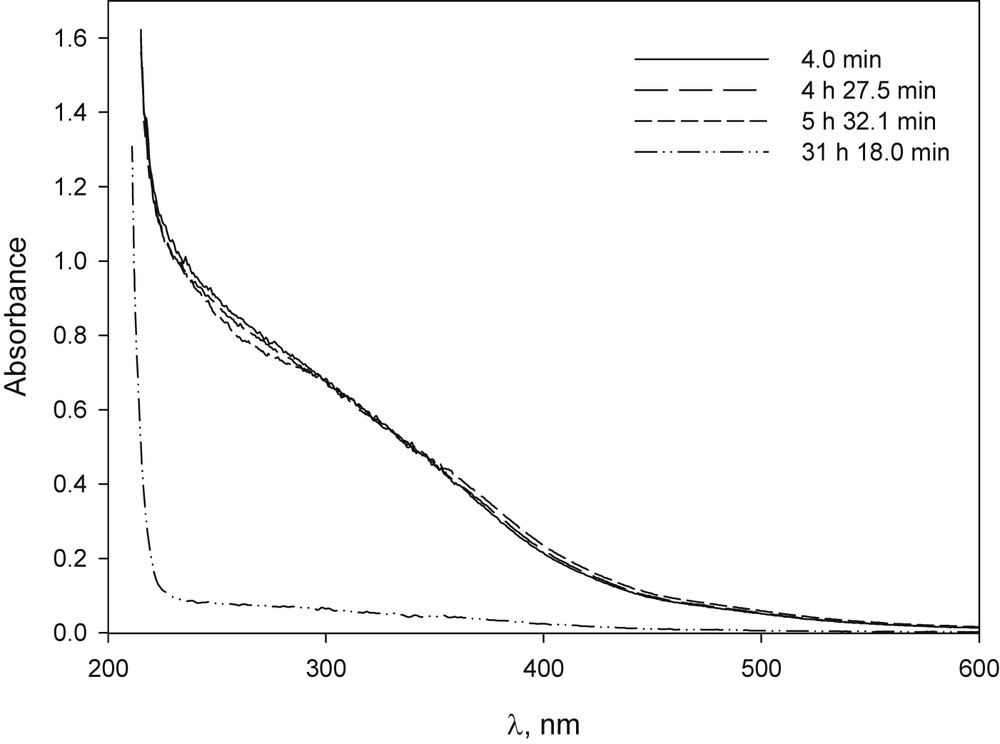
UV–vis spectrum of FeBr3 in NaOH solution at different time after preparation: [FeBr3] 2.4 × 10−4 M, [NaOH] 1.0 × 10−2 M.
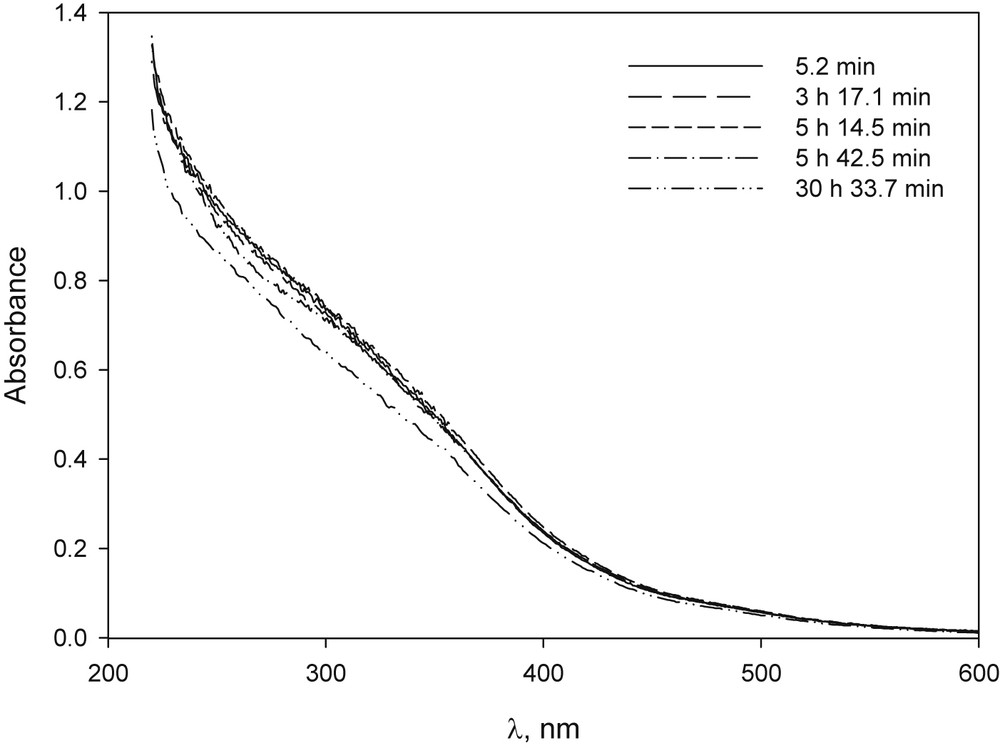
UV–vis spectrum of FeBr3 in NaOH solution in the presence of β-CD solution at different time after preparation: [FeBr3] 2.3 × 10−4 M, [β-CD] 1.7 × 10−4 M, [NaOH] 1.0 × 10−2 M.
Similar studies were carried out with ferric chloride and nitrate salts. Ferric chloride showed a similar behaviour to that of ferric bromide (Fig. 7S). Ferric nitrate remained, in comparison with other salts, more stable over time (Fig. 9S). In all cases, in the absence of CD, after 5 h, the presence of precipitate was clearly observed. When the metallic salts were dissolved in a basic solution containing β-CD, at a concentration similar to that of the metal centre, no precipitate was observed in the period studied and the spectrum was very similar to that of ferric chloride (Fig. 8S). With the ferric nitrate salt, the initial and final UV–vis spectra were virtually the same (Fig. 10S).
Studies of FeBr3 at pH 7 carried out in the presence of an imidazole buffer showed immediate precipitation of iron in the absence of β-CD, whereas in the presence of the oligosaccharide a precipitate was observed after 10 min of mixing.
3.3 Cobalt
Cobalt compounds have a variety of industrial applications, among them as catalysts. It is also known that some salts such as CoCl2 can interact with saccharose [50].
Stability studies were performed with cobalt(II) bromide, chloride and nitrate in the presence and absence of β-CD and at different CD/metal molar ratios. In most cases and during the first few hours after the dissolution, an increase in absorbance was observed at wavelengths less than 400 nm. This could indicate the presence of a new species and/or geometric isomers that could also generate colour change in the aqueous solutions. In general, at the end of the period studied, the absorbance decreased completely and a precipitate was observed.
In the case of CoBr2, when it was dissolved in basic medium, a blue precipitate was immediately formed, probably corresponding to the hydroxide Co(OH)2, which then turned brown due to the formation of Co(OH)3 (Fig. 11S). This oxidation is attributed to the decrease in the potential Co(III)/Co(II) in basic medium in the presence of atmospheric oxygen [38]. The β-CD/CoBr2 molar ratios used were about 1/1, 5/1 and 35/1 and precipitation was retarded but it could not be avoided. The colour of the solution changed from purple to green and finally to yellow (Figs. 5 and 12S).
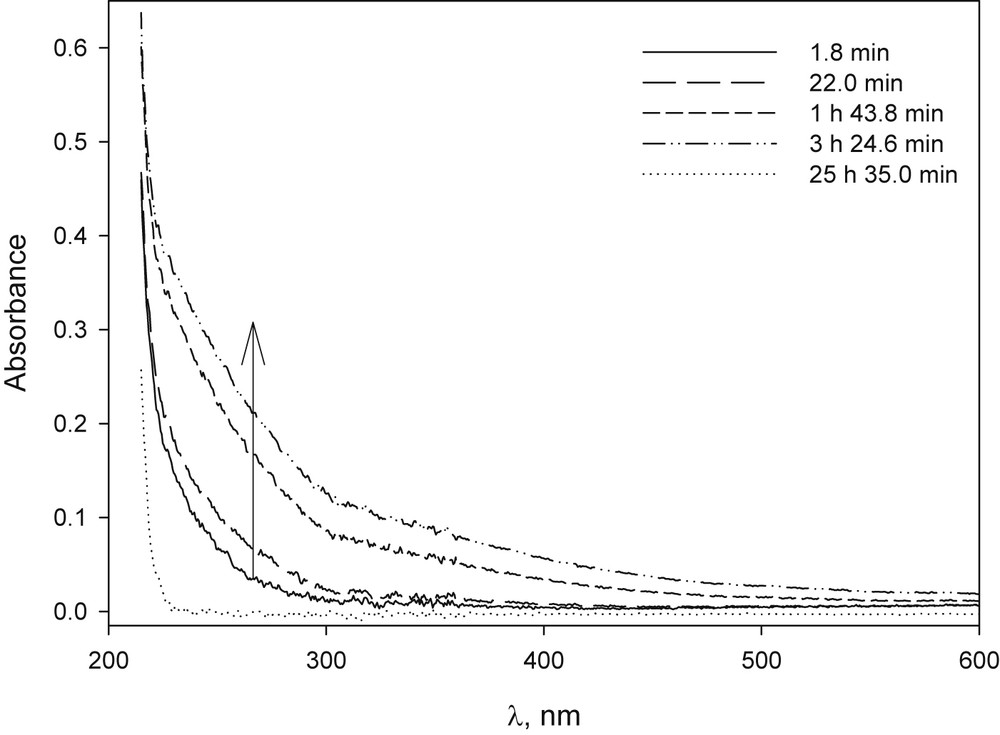
UV–vis spectrum of CoBr2 in NaOH solution in the presence of β-CD solution at different time after preparation: [CoBr2] 1.6 × 10−4 M, [β-CD] 1.8 × 10−4 M, [NaOH] 1.01 × 10−2 M. The arrow indicates the increase in absorbance observed at the beginning of the study.
When cobalt(II) chloride was studied in basic medium, a precipitate was observed, its colour changed from light blue to green and finally to brown (Fig. 13S).
Studies were then conducted at four CD/metal molar ratios: 1/1, 5/1, 10/1 and 20/1 approximately. A precipitate was observed in the first three cases but, as the relative concentration of CD increased, its appearance was delayed. In all cases, an increase in absorbance was observed, indicating the presence of a new species that would involve β-CD (Fig. 14S) because this effect was not observed in its absence. The behaviour is similar to that found with cobalt bromide. When the ratio was 20/1, during the study period there was no precipitation; yet, the solution that was initially purple, after 3.50 h, turned green and finally translucent yellow-green.
Cobalt(II) nitrate showed an intermediate behaviour as compared to that of other salts. In basic medium, it formed a light blue precipitate, which after a few minutes changed to brown (Fig. 15S). In the presence of CD, 1/1, the solution was translucent purple, and after a few hours, a green-yellow precipitate was formed. In both cases the absorbance increased in a first stage and then decreased (Fig. 16S).
3.4 Nickel
Nickel is a 2+ cation in their common compounds, but also can be found in oxidation states 0, 1+, 3+, 4+. Nickel forms a variety of coordination complexes and simple compounds or salts [39,51]. Most nickel species are green or blue due to hydration or binding of other ligands to the metal centre. The nickel ion present in aqueous solutions of single compounds is itself a complex [Ni(H2O)6]2+ [38].
Studies carried out on nickel(II) bromide, chloride and nitrate showed a low capacity of β-CD to stabilize the metal salt in basic medium (Fig. 6). Precipitation was not avoided using β-CD/Ni(II) 1/1, 5/1 and 12/1 ratio. A brown precipitate was formed in all cases; however the rate of formation decreased with an increasing concentration of CD (compare Figs. 7 and 17S).
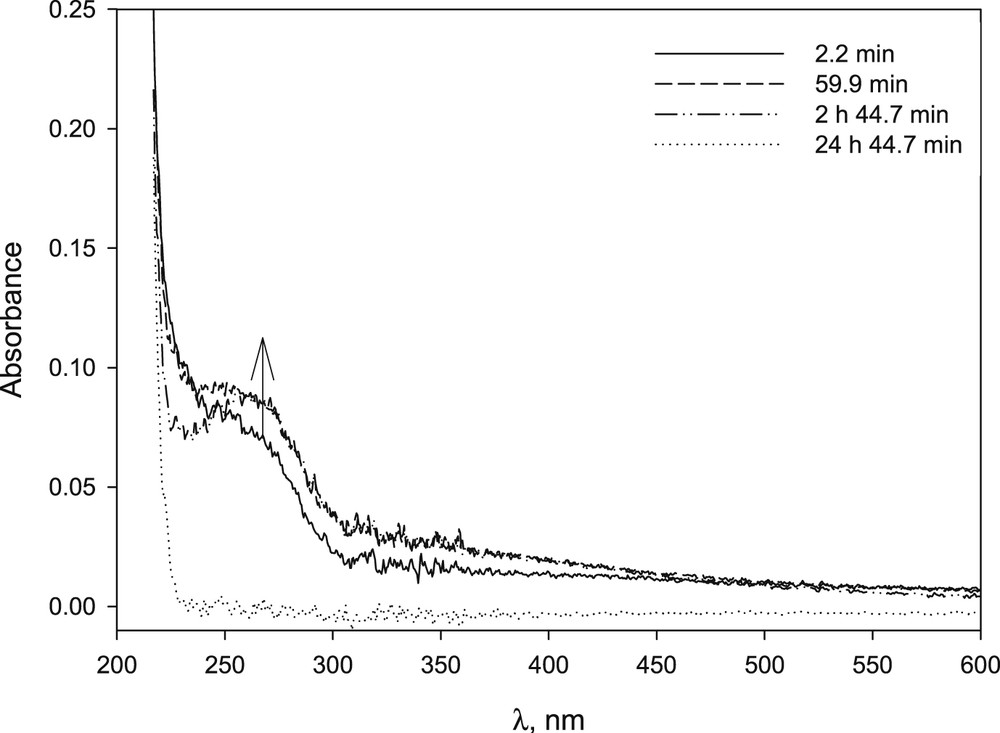
UV–vis spectrum of NiBr2 in NaOH solution at different time after preparation: [NiBr2] 1.9 × 10−4 M, [NaOH] 1.01 × 10−2 M. The arrow indicates the increase in absorbance observed at the beginning of the study.
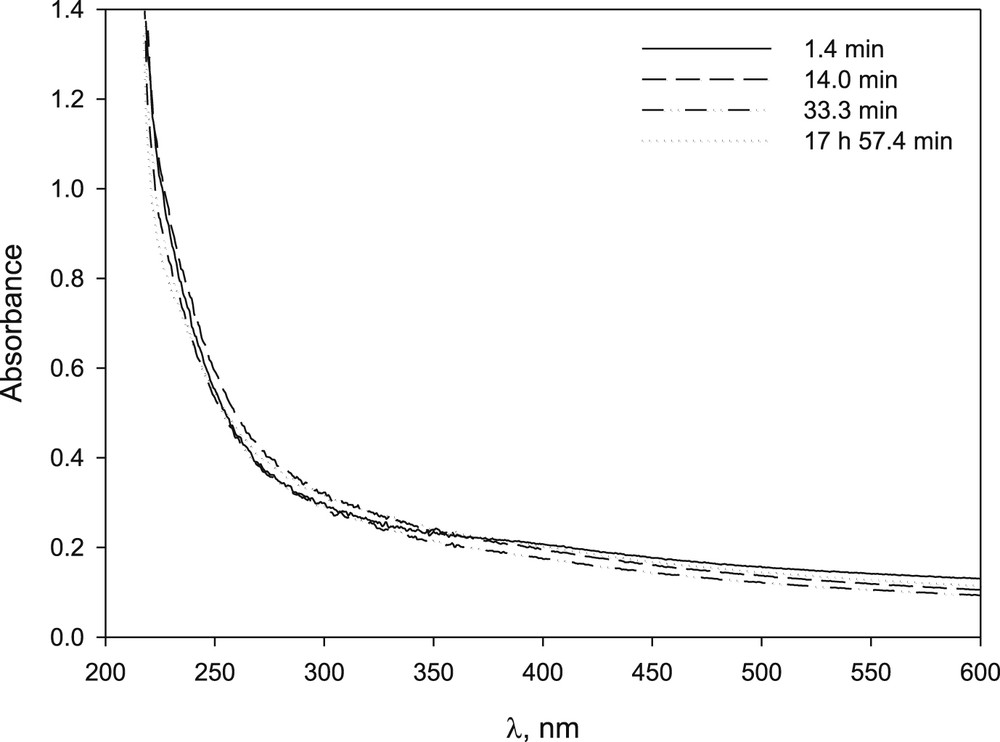
UV–vis spectrum of NiBr2 in NaOH solution in the presence of β-CD solution at different time after preparation: [NiBr2] 8.2 × 10−4 M, [β-CD] 9.98 × 10−3 M, [NaOH] 9.94 × 10−3 M [β-CD]/[NiBr2] = 12.2 ratio.
NiCl2 behaviour was similar to that of the bromide in basic medium, a light green precipitate was formed immediately (Fig. 18S). With the addition of increasing amounts of CD, a brown precipitate was slowly formed. In the study at CD/salt 1/1 molar ratio, the behaviour was identical to that in the absence of β-CD, showing a continuous decrease in absorbance (Fig. 19S). However, solution with 10/1 and 20/1 molar ratios showed a slight increase in absorbance at values lower than 400 nm during the first few hours of the study (Figs. 20S and 21S).
When nickel nitrate was studied, an immediate precipitation was observed in basic medium and in β-CD/salt 1/1 molar ratio (Figs. 22S and 23S), but when the ratio was 5/1, no precipitation was observed and the shape of the spectrum and the absorbance values changed (Fig. 24S).
3.5 Copper
In a recent report, it was proposed that in solid complexes of β-CD–CuCl2, the presence of β-CD molecules weakens the strength of ionic bonds in CuCl2 by molecule–ion interaction with the Cu2+ and Cl− ions [52]; Cu complexes with a chitosan derivative were also synthesized [53] and sorption of Cu2+ ions into the cellulose matrix was found [54].
In previous work, we determined that CuBr2, CuCl2 and Cu(NO3)2 form stable solid complexes with α, β and γCD. The procedure for their preparation is simple and yields complexes with 1/1 stoichiometry containing also water molecules. Electron paramagnetic resonance (EPR) and reflectance measurements showed that the complexes have octahedral coordination geometry [55].
The studies were carried out with copper(II) bromide, chloride and nitrate and different β-CD/metal salt molar ratio. Copper(II) bromide in basic medium and the solution containing a β-CD/metal 1/1 molar ratio precipitated immediately, with a marked decrease in absorbance values (Figs. 25S and 26S). However, when the ratio was 10/1, the solution showed a very faint blue colour and neither precipitate nor significant decrease in absorbance was observed during 27 h (Fig. 27S).
In basic solution, the CuCl2 behaviour was different from that of copper(II)bromide because a light blue precipitate was observed, which eventually turned brown (Fig. 8). In the presence of β-CD and when the ratio was 1/1, a light blue translucent solution with no precipitate was observed during the time studied, with only a slight variation in absorbance (Fig. 9). Cu(NO3)2 showed a similar behaviour (Figs. 28S and 29S).
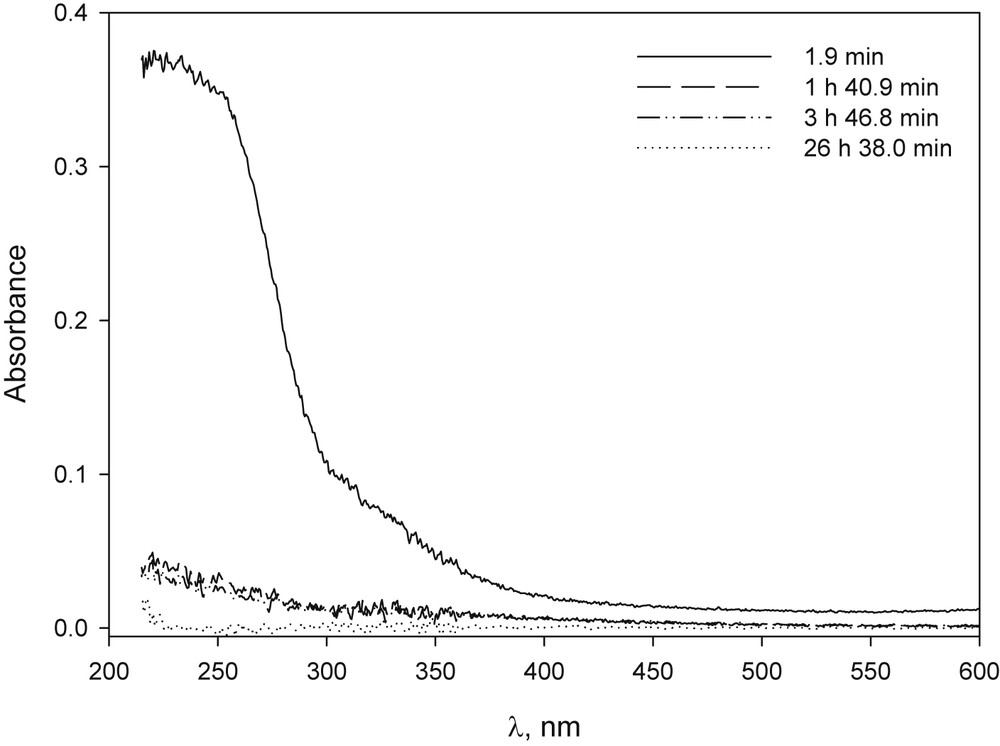
UV–vis spectrum of CuCl2 in NaOH solution at different time after preparation: [CuCl2·2H2O] 1.6 × 10−4 M, [NaOH] 1.0 × 10−2 M.
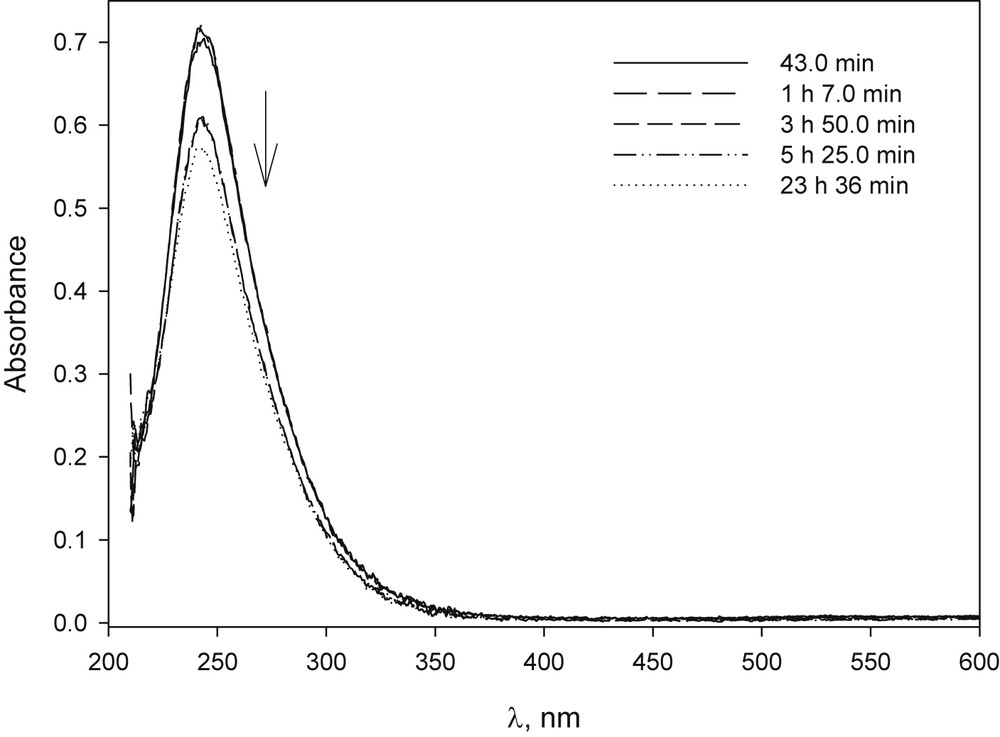
UV–vis spectrum of CuCl2 in NaOH solution and in the presence of β-CD solution at different time after preparation: [CuCl2·2H2O] 1.0 × 10−4 M, [β-CD] 1.0 × 10−4 M, [NaOH] 9.9 × 10−3 M.
3.6 Zinc
Zinc is always divalent in its compounds, except when it joins some other metals, forming alloys of zinc. It also forms many coordination compounds where the fundamental structural unit is a central zinc ion, surrounded by four coordinated groups spatially arranged in the corners of a regular tetrahedron [38,39].
Studies were only conducted with zinc(II) bromide. When this salt was dissolved, it showed no electronic spectrum in the UV–vis region that would enable monitoring the reaction as with other metals, probably by their electronic configuration [Ar]4s23d10. However, in the early hours, a minimum increase in absorbance at about 260 nm was observed, which then disappeared completely; this could be due to the formation of some complex or species in the basic media even in the presence of CD (compare Figs. 30S and 31S) [56]. A similar precipitate was formed in the presence and absence of CD, indicating that CD did not stabilize the salt in solution.
Because we used UV–vis spectroscopy to control the stability of a solution and in the case of Zn there was no absorption in the useful range of the spectrum, no further studies were carried out with Zn salts.
3.7 General comments
It was reported that the formation of β-CD complexes with Cu2+ [25] requires deprotonation of β-CD. CuCD(OH)22− complex is formed in alkaline solution (11 < pH < 14) in the presence of excess of β-CD [57]. On the other hand, we have previously shown [36] that in the nonaqueous solution FeBr3 forms complexes with β-CD. In this case no ionization is required, indicating that the OH groups of CD can coordinate with the metal ion even in its neutral form.
In the study reported here, the salts are dissolved in basic water and the interaction of the metal with CD has to compete with other reactions. As example, reactions (1)–(4) are shown; however, it should be kept in mind that there are other possible reactions in solutions of transition metals [58].
(1) |
(2) |
(3) |
(4) |
It is likely that reaction (4), which involves CD anion (CDO−), is more efficient to compete with the other possible reactions. In addition, according to data mentioned above, K3, which involves the interaction of neutral CD (CDOH) with the metal, should be smaller than K4. On the basis of the literature data [25,57], β-CD pKa value is 12.2 [17], therefore, 39% of β-CD concentration is found as β-CDO− anion at [NaOH] 1.0 × 10−2 M. Then, reaction (4) should predominate under the condition of this study.
The competition among reactions (1)–(4) can partially explain the different efficiency of CD to stabilize the metal salt solutions. In addition, it is important to take into account that the stabilization of complexes depends also on the metallic centre and the counterion. A high value for equilibriums (3) and (4) also depends on the geometry of the complex formed with CD and it is likely that the bigger size of Zn turns the interaction with metal less efficient. For CD to stabilize the metal in solution, equilibrium (4) must be more efficient than (1), since the precipitation of the metal is produced by reaction (1) and all the reactions derived from M(n−1)+(OH).
The competition among reactions (1)–(4) is evident from the following facts:
- (1) The anion of the salt is very important to keep or not the metallic centre in solution. Thus, in this respect, NO3− appears as the best anion.
- (2) The anion of CD is a coordinating agent better than CDOH; as a consequence, when the experiment was carried out at pH 7 with imidazole as buffer, FeBr3 precipitation was slightly retarded but not prevented.
It is important to note that, in the presence of imidazole (Im), in addition to equilibriums (1)–(4), reaction (5) should be considered [59]. Therefore in those experiments, not only ionized CD is absent but also the concentration of free CD decreased due to equilibrium (5).
(5) |
MnBr2 and MnCl2 salts were stabilized for about the first 6 h in the presence of β-CD, whereas in the absence of the oligosaccharide, precipitation was immediate. Moreover, when Mn(NO3)2 was used there was no precipitation in 24 h. This indicates that a different interaction between counterions (chloride, bromide or nitrate) and the metallic centre can be found as was reported in other studies [60,61].
The results obtained with Fe3+ showed an excellent interaction of β-CD with the metal centre, in agreement with data reported in the literature regarding the interaction with d-gluconate [62] or in ternary complexes with 2-naphthol and caboxymethyl-α-CD [63]. The relative stability of the salts in aqueous solution, in the absence of the β-CD, follows the order reported in the literature, FeBr3 < FeCl3 < Fe(NO3)3, and is consistent with the affinity of these ions for the coordination to Fe(III) [64–66].
With cobalt, the trend of the counterions is the same, cobalt bromide could not be stabilized under any condition, whereas when the β-CD/Co(II) chloride ratio was approximately 20/1, precipitation was prevented. Finally, Co(NO3)2 did not precipitate during the early hours of study in the presence of β-CD. Considering the colour of the precipitate and/or the solution the presence of β-CD did not seem to prevent the oxidation of Co(II) to Co(III).
In the case of nickel, only the nitrate could be stabilized, whereas copper salts had similar behaviour to that of iron; only copper(II) bromide needed a higher ratio to be stabilized. In particular, copper salts in the presence of CD showed a significant band at 250 nm.
Table 1 summarizes the stability of different metallic salts in NaOH 1 × 10−2 M solutions with or without β-CD/metal salt ratio of 1/1.
Qualitative stability studies of transition metal salts in NaOH 1 × 10−2 M, with equal molar amounts of β-CD during 24 h.
Anion | Mn2+ | Fe3+ | Co2+ | Ni2+ | Cu2+ | |||||
β-CD | – | β-CD | – | β-CD | – | β-CD | – | β-CD | – | |
Br− | × | ● | × | ● | ● | ● | ● | ● | ● | ● |
Cl− | × | ● | × | ● | ● | ● | ● | ● | × | ● |
NO3− | × | × | × | × | × | ● | ● | ● | × | ● |
Considering the results, it can be said that the affinity of the metal centre by β-CD or its anion under the conditions studied increases in the order:
The major stability of the Fe3+ complex as compared to that of divalent metal complexes is expected on the basis of the charge and ionization potential of the metal ions; Fe3+ is also electronically equivalent to Mn2+. On the other hand, the behaviour of other metal centres does not correlate with their periodic properties. Different factors such as affinity by donor oxygen, coordination capacity and hydrolysis should lead to the order observed [67,68]. For example, although with the increase in the atomic number of the metallic centres a higher molar ratio of βCD/metal centre was necessary; copper did not follow this tendency, presenting a behaviour similar to that in iron. On the other hand, the counterions followed the order of the spectrochemical series in stability of salt and in the complexes that could be formed with β-CD, as well
In some cases, the presence of β-CD produced large increases in absorbance as compared to a basic solution of similar concentrations. This fact may be related to the formation of complex species, able to remain stable in this medium.
Many of these solutions also show variation in the colouration with time. This observation could be due to an efficient competition of the hydroxyl groups of β-CD with water and/or hydroxyl group for the metal centre, which avoids formation of hydroxides. Nevertheless, the presence of this ligand would not significantly change the oxidation potential of the metallic cations, where oxidation can occur.
With the data available, it is difficult to propose structures for the complexes that could be formed in each case, but it is likely that they are similar in nature to those proposed in the references indicated above. The only coordinating atoms in CDs are the oxygens, located in the cavity (glycosidic oxygens) and at the rim (primary or secondary OH groups), but it is not possible to determine a preferred position for the metal centre. However, it can be assumed that a higher orbital overlap is most favoured as the guest is more included, its mobility is constrained in a confined space, so the interaction may be more efficient [69]; in this position, the metallic centre would be more protected from the basic medium.
Several isomers of the complex may be present in the solution. This is possible because more than one geometry would be allowed for a big ligand with multiple coordinating atoms such as CD. Even with identical stoichiometry, four different ligands (halide or nitrate, oxygen from β-CD, oxygen from water molecules and/or oxygen from hydroxide ion) may be involved in the coordination process and differences in the coordination position (equatorial, axial or both) render M2+ or M3+ atoms with different environments. For illustrative purposes, Scheme 1 shows some of the coordination possibilities for a general complex of β-CDMX2 or 3(H2O and/or OH−)n. For the same position of the metal atom, structures (a–c) show different coordination possibilities, where axial position may be occupied by different ligands (a and c) or the same (b); the counterion can be in equatorial position (b) or in a combination of equatorial–axial inside or outside the cavity (a and c). Schemes from (d) to (f) show some of the possible coordination for M2+ or M3+ with the oxygen atoms of CDs, where only one glucose unit may be involved (d or e) or metal centre could bind two different ones (f); the coordination may involve secondary OH (e), glycosidic O (d) or combination of these.
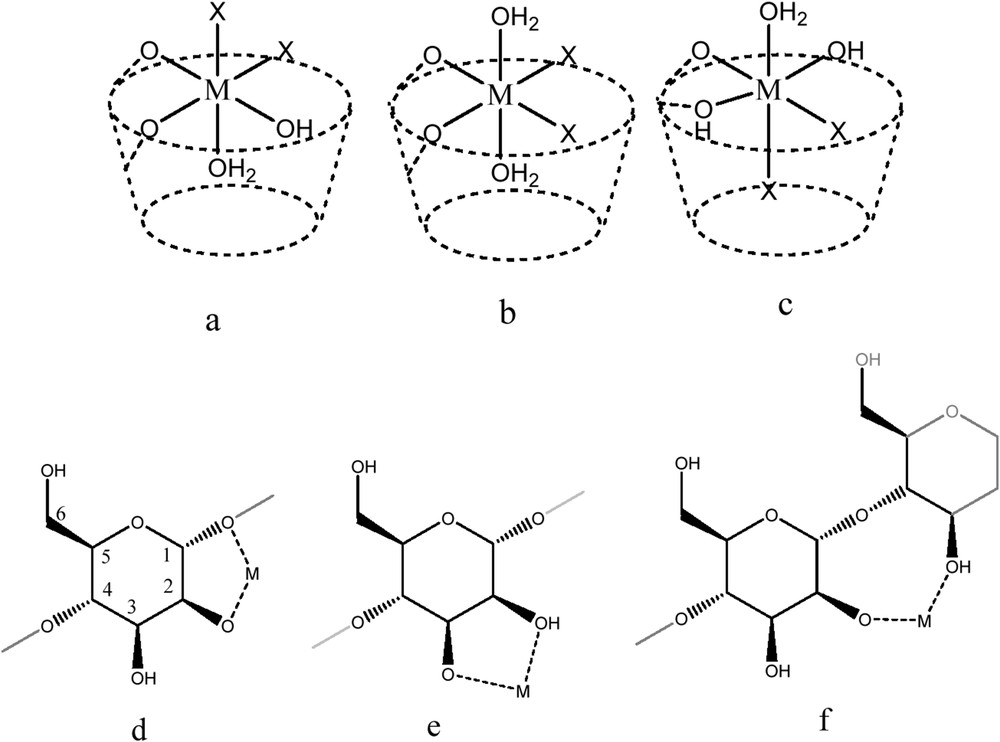
Schematic representation of different coordination possibilities (possible isomers) for a M(II) or M(III) metal centre with the same ligands in its first coordination sphere but in different arrays (no scale).
The results reported here show that Mn, Fe, Cu and Co can remain in solution in basic water when they are in the presence of β-CD.
4 Conclusions
We found conditions under which hydrolysis and subsequent precipitation of various metal salts of the first transition series were avoided. The optimal stabilization conditions changed not only with the metal cation but also with the counterion present.
The CD concentration required to avoid precipitation of metallic centres is different for each one. No correlation was observed with periodic properties. It was established that salts with bromine counterion showed a lower tendency than others to be stabilized in the presence of CD.
Besides, a better stabilization of the metal ion was obtained when nitrate metal salts were dissolved in β-CD–NaOH solution.
Acknowledgements
L.I.R and R.H.R. are researchers from Consejo Nacional de Investigaciones Científicas y Técnicas (CONICET). C.O.K. was a grateful recipient of fellowship from CONICET. This research was supported in part by CONICET (Nº5796), Agencia Nacional de Promoción Científica y Técnica (FONCYT) (Nº217/06), Secretaría de Ciencia y Técnica (SeCyT-UNC) of Universidad Nacional de Córdoba (Nº162/06) and (Nº114/07) and Ministerio de Ciencia y Tecnología de Córdoba (MINCyT) (Nº1210/07), Argentina.