1 Tutorials on the fundamental aspects
1.1 Introduction on spin crossover
When one searches for the literature on Fe(II) or Fe(III) spin-crossover (SCO) systems, several excellent books and reviews appear giving an overview over the wide field of different ligand types that can be used for the synthesis of such complexes [1–8]. At a first glance, this is surprising, as the preconditions for the observation of an SCO without a change in the coordination number (other possibilities to switch the spin state with a change in the coordination number are referred to as coordination-induced spin-state switch [CISSS]) are actually quite limited. First of all, an octahedral coordination sphere is usually required for the observation of this phenomenon and exceptions from this rule are very rare. So far only few examples with coordination number 4 [9,10] and 5 [5,11–13] (besides iron porphyrin and heme) [14,15] are known. However, this is clearly not the limiting factor, as the coordination number 6 is probably the most frequently one observed since the foundation of coordination chemistry by Alfred Werner. In agreement with this, for all the different ligand combinations leading to coordination number 6 at least one example for a [FeIIN6] SCO complex is known. In Fig. 1, the different possibilities are given with a representative example for each.
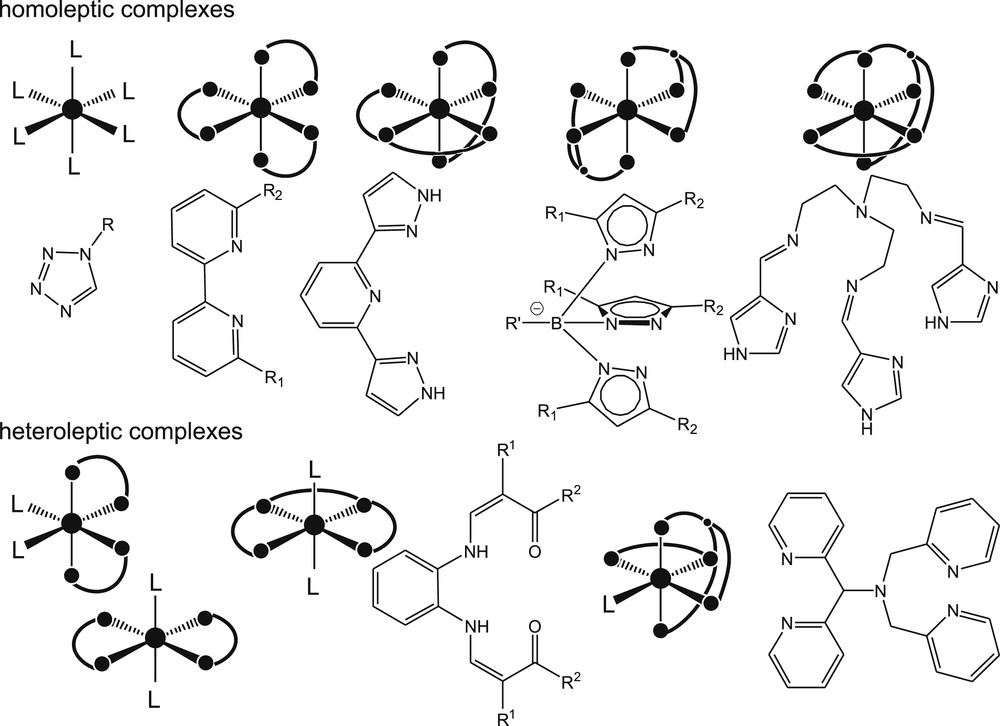
Possible ligand combinations for the synthesis of octahedral ferrous complexes with SCO properties.
Starting with homoleptic complexes, as monodentate ligands 1-alkyl-tetrazoles were successfully used for the synthesis of SCO complexes [3,16]. Derivatives of 2,2′-bipyridine (bipy) or 1,10-phenanthroline (phen) are often used as bidentate ligands [17]. Furthermore, a series of bidentate ligands for homoleptic SCO compounds are known [1,18], where different N-heterocycles are combined in one ligand, for example, pyridine and triazole or where primary amines and suitable aldehydes are combined with Schiff base type diimine systems. In the case of tridentate ligands for homoleptic ferrous SCO complexes, the spectrum is even wider. Next to various combinations of N-heterocycles (terpyridine, bpp) [1] that serve as meridionally coordinating ligands, the trispyrazolylborate/-methanide/-methane–based ligands that coordinate facially are suitable middle field ligands [2]. In addition, a wide spectrum of tridentate Schiff base ligands, often with an N2O donor atom set, are highly suitable for the synthesis of homoleptic ferrous SCO complexes [8]. Heteroleptic SCO complexes can be obtained by a combination of slightly stronger field ligands like bipy, phen, or 2,2′-bipyrimidine, where one or two of the molecules are replaced by weaker field ligands (mostly monodentate) like pyridine or NCS−. Alternatively, combinations of tetradentate pyridine-based, Schiff base, or Schiff base–like ligands with suitable monodentate ligands are very frequent [4,7]. Examples for pentadentate ligands (combination of aliphatic and aromatic nitrogens) are relatively rare [4]. Here, the spin state strongly depends on the sixth ligand. Hexadentate ligands suitable for the observation of SCO in ferrous complexes are branched chelate ligands obtained through a combination of aliphatic and aromatic nitrogens, Schiff base ligands with imines as donor functions, or Schiff bases with N4O2 donor set [4].
A common feature of all those ligands is that they are middle–strong field ligands (σ-donor ligands). The similar ligand field strength of all those ligands is, for example, reflected in the similar color of many of the Fe(II) complexes, provided they have identical chromophores. For example, azole-type derivatives are always red in low-spin state and colorless in the high-spin state. In principle, there are two possibilities to tune the ligand field strength to reach the region where SCO is observed. The first option is to control the FeL bond lengths through steric effects as ΔO strongly depends on the FeL distance. This concept is very successfully applied to complexes with bipyridine-, phenanthroline-, terpyridine-, and trispyrazolyl-based ligands where, depending on the position and steric demand, bulky substituents reduce or increase the FeL bond length [1,2]. However, this concept also works for N2O22− coordinating Schiff base–like ligands. Here, the replacement of the phenylene bridge by an ethylene bridge leads to pure high-spin Fe(II) complexes due to an elongation of the FeN bond lengths [13]. If only the donor strength of the nitrogen would be considered, an opposite result would be expected. The second possibility is the use of electron withdrawing or donating substituents that control the σ-donor strength of the donor atoms. Because of the higher positive charge of Fe(III) compared to Fe(II), the ligand field splitting ΔO is expected to be larger in the case of Fe(III) complexes, if the same ligands are used. However, as the spin pairing energy is much higher in the case of Fe(III) [19], no general statements can be made for the impact of a mere change in the oxidation state at the iron center on the spin state of the complex. The higher ligand field stabilization, which is required for the SCO of Fe(III) complexes, is usually obtained by introducing negatively charged chelating ligands.
SCO phenomenon can be observed in solution as well as in the solid state. In the latter, gradual or cooperative SCO can be observed. The term spin transition (ST) refers to cooperative SCO without or with hysteresis. The hysteretic behavior reveals a first-order phase transition. In solution, interactions between the SCO centers are negligible and the SCO follows a temperature-dependent Boltzmann distribution, so a very gradual SCO is observed. In the solid state, some systems also show gradual SCO; however, abrupt SCOs are also observed where long-range elastic forces between the transiting molecules lead to a cooperative ST (electron–phonon coupling). The effective communication of the volume change during the ST from one molecule to another was discussed for a very long time as a highly important aspect, for example, in the model of internal pressure developed by Spiering and co-workers [20]. It proposes that the volume changes can be considered as point defects that lead to an internal pressure, which then expands through the crystal lattice via phonon interactions, triggering the spin-state change at the other SCO centers.
There are many different means to realize an effective communication of these structural changes. One possibility is rigid covalent linkers that allow a relatively easy control over the dimension of the network [21]. Systematic investigations on the ST behavior of one-dimensional (1D) coordination polymers in combination with results of theoretical simulations reveal that rigid linkers in combination with additional interchain interactions are necessary for the observation of cooperative effects [22]. Wide hysteresis loops can also be observed for mononuclear complexes if only intermolecular interactions are present, as, for example, van der Waals interactions [23], π–π interactions [24], or hydrogen bonds [25]. In 2011, Halcrow [26] initiated a new concept, which combines the input of different theories to explain cooperativity and wide hysteresis loops. He suggested that not only volume changes but any structural changes upon ST can be responsible for cooperative interactions. These include the rotation of ligands or structural changes in the orientation of side groups. All these changes can serve as an energy barrier for the transition from one spin state to the other and thus lead to different transition temperatures upon cooling and heating. With this concept, the observation of cooperative ST in complexes with relatively soft amphiphilic ligands can be explained [27]. An excellent summary of complexes with very wide hysteresis loops is given by Brooker [28]. In the case of Fe(III) complexes, the volume change upon SCO is smaller and the observation of ST with hysteresis is less frequent [8]. Nevertheless, some systems with wide hysteresis due to π–π interactions are known [29].
1.2 Interest and first developments toward applications
As early as 1988, Kahn and Launay [30] envisioned the exciting perspectives of molecular electronics based on molecular bistability. One route they proposed for high-density storage devices is based on the design of molecular assemblies or polymers exhibiting cooperative ST with a room temperature (RT) hysteresis, the nanoscale processing of bistable objects, and also the integration of functional units in genuine devices [31,32].
This proposition that emerged with the demonstration of the robust bistability around RT of 1D Fe(II) triazole polymers has represented an important milestone in the development of the domain [33]. It followed a strong activity directed toward potential applications of bistable materials [34] in parallel to the search for new cooperative and hysteretic materials. For the latter, the dimensionality and intersite interactions (nature, strength, and directionality) were varied as detailed above, via the design of 1D–3D coordination polymers. With respect to molecular materials, both their intrinsic structural versatility (structure derived from supramolecular interactions) and their limited chemical stability render them a bit less attractive for implementing genuine applications in information storage. However, a relatively rich literature witnesses of the significant development of new Fe(II) molecular solids that undergo cooperative transformation centered at RT [35], with wide hysteresis [36]. Examples of Fe(III) analogs [5,37] exhibiting RT bistability or multistability still correspond to a minority [8].
The discovery of light-induced excited spin-state trapping (LIESST) in 1984 [38] has represented an important breakthrough with respect to the knowledge of the mechanism and dynamics of the photoinduced processes [39]. It gave rise to new areas of research centered on the photoinduced phase transition in solids and the applicative developments of photoswitchable functionalities. The use of light, a rapid, easily tunable, and noninvasive stimulus, makes it possible to reversibly control the spin-state switching in bulk nanomaterials and ultimately at the molecular level. The ST induced by the LIESST effect requires a sufficiently low temperature (T < 50 K) for slowing down the relaxation from the metastable high-spin (HS) state to the low-spin (LS) ground state. The competition between photoexcitation and thermal relaxation has been exploited for promoting a photoinduced hysteresis by cycling of the temperature in the presence of continuous irradiation (light-induced thermal hysteresis) or the intensity sweep rate at a fixed temperature value (light-induced optical hysteresis).
The photoinduced bistability of materials is a key feature for the exploitation of all-optical data storage and processing devices, but to be realistic, this application requires higher working temperature, typically close to ambient one. The temperature range in which the lifetime of the S = 2 state is long enough for detecting the photomagnetic response has been investigated with the so-called TLIESST approach [40] (TLIESST being defined at the inflection point of one peculiar curve of the LIESST relaxation). A wide database, gathering the values of TLIESST and T1/2 (transition temperature for the thermal SCO), has been established with several molecular materials. From that, trends showing a correlation between the nature of the coordination sphere of the metal and the lifetime of the photomagnetic state have been proposed as a guideline for the design of long-lived photoactive materials. Increasing the temperature above TLIESST reduces the lifetime of light-induced HS species. An application of this characteristic was the creation of laser-induced dynamic gratings in an LS material, which demonstrates potentialities for real-time holographic data processing [41].
To observe long-lived spin states at ambient temperature, a physical strategy exploits the single-shot photoswitching inside the hysteresis of a bistable material, which results, at least partly, from the laser heating [42]. Moreover, chemical strategies use the coupling between SCO and a secondary process. In the ligand-driven light-induced spin change (LD-LISC) effect [43,44], the structural change of a photoisomerizable ligand modulates the ligand field strength that controls the spin state of the metal ion. Introducing ligands exhibiting a thermally irreversible isomerization (Z/E isomerization [45], electrocyclization [46,47]) allows the spin-state switching to be controlled by light and to target at the elaboration of reversible optical memories at the molecular scale. The structural rearrangement determines the feasibility in the solid state, whereas the chemical stability is mandatory for applications in solution. Theoretical analyses are required to describe electronic, structural, or energetic parameters of LD-LISC type complexes or the electronic excitation of the photoactive ligand.
Moreover, the configurational relaxation [48–53] of n coordinate SCO compounds (with n = 6 or 7) that occurs from the light-induced HS excited state suits for opening slower deactivation pathways toward the LS ground state. Beyond the SCO of 3d4–3d7 octahedral complexes, the light-driven CISSS strategy uses the changes in the coordination number of a Ni(II) complex in solution to drive the reversible switching between the diamagnetic and paramagnetic states. The Z/E photoisomerization of the diazopyridine attached to the ligand skeleton controls the coordination of the pyridine ring to the square planar Ni(II) complex forming a square pyramidal complex. These photoactive molecules were proposed for applications in magnetic resonance imaging [54,55].
1.3 Multifunctionality
As introduced above, a fertile research field directed toward applications explores the multifunctionality resulting from the assembly of two molecular fragments—one of which being SCO type—that operate in synergy under stimulation to achieve an external control. The present developments include the combination of SCO and electronic functionalities (conductivity, magnetism, or optic), the formation of peculiar structural arrangements (self-assembly), or architecture (for instance with porosity). For that, a multiplicity of properties have been targeted as mesogen, sorption, molecular recognition [56], magnetic exchange, conductivity [57,58], luminescence, electroluminescence [59,60], nonlinear optics [61], chirality [62], ferroelectricity, or redox. The synthetic strategies use either the self-assembly method (template effect, dispersion, or electrostatic forces) or the postsynthetic approach (counterion or ligand exchange). The switchable character of the SCO type unit within the molecular assembly may depend on the conditions adopted for the chemical synthesis. Nevertheless, several multifunctional materials have been successfully elaborated, which exhibit the expected synergies because of the coupling of functions or the coexistence of the latter [63]. Different reviews covering this area up to 2012 are found in Halcrow's book. Murray [63] described the main families of multifunctional SCO materials. By addressing the issue of the SCO phenomenon in soft matter, Gaspar and Seredyuk [64] treated various systems (such as lipidic molecules, gels, liquid crystals, dendrimers, and Langmuir–Blodgett films) from which they discussed the synergetic effects between SCO and peculiar structural organization. Shepherd et al. [65] focused on the combination of SCO with luminescence together with the nanoscale applications of hybrid SCO materials. Lacroix et al. [66] provided an overview of multifunctional materials and molecular switches combining the nonlinear optics and the multiple functionalities derived from coordination chemistry. Sato et al. [67] summarized the research on both, the SCO conductors and the SCO magnets. The guest effects on SCO in metal–organic framework, first reviewed by Muñoz and Real [68], were nicely updated by Ni et al. [69]. With respect to the emergence of switchable molecular spintronic devices, Ruiz [70] treated the issues of the charge transport properties in thin films, nanoparticles, and ultimately single molecules. Lefter et al. [71] proposed a review on electrical and charge transport properties of SCO complexes and the related devices. A concise overview of Kumar and Ruben [72], dedicated to the emerging trends in SCO-based functional materials and devices, discusses the recent advances in electrical and spin transport characteristics of thin film SCO junctions as well as the hybrid materials and the solvent sensors.
1.4 Processing
With respect to the technological perspectives, the processing [73] of SCO objects or their integration in nanodevices [74] represents a critical point as it implies to maintain and control the properties at the relevant scale or in a peculiar environment. The molecular compounds existing in crystalline modifications [26,75] being discarded because of their variable SCO features, the stability criteria is in favor of strong chelates of SCO Fe(II) [76] or Fe(III) [77] metal ions. Several wet approaches suit for processing at nanoscale highly soluble compounds: precipitation with an antisolvent, precipitation and confinement controlled by the spin coating on silica. Precisely, the spin coating of a dispersion of particles within silica or an organic polymer allows the fabrication of films with easily tunable characteristics. It is notable that the polymers used as confining, dispersing, or stabilizing agent may play an active role—the so-called matrix effect—manifested by the spreading and the shift of SCO or the opening of a hysteresis loop [78]. The case of Fe(phen)2(NCS)2, an electrically neutral and thus poorly soluble compound, is especially informative as various approaches were developed for the processing. The nanoparticles formed by the reverse-micelle technique or the solvent-assisted precipitation exhibit either a hysteresis, which is larger than the bulk one, or a gradual SCO [79], a finding showing—among other things—the importance of crystallinity on the SCO properties in molecular materials. A lithographically controlled wetting method leads to the nano/micropatterning over large areas of the SCO compound found in a new crystalline form [80]. Thin films may be obtained by the Langmuir–Blodgett technique that consists of the sequential deposition of monolayers [81] but both the functionalization of ligands with alkyl chains and the processing stage can modify the SCO features [82] The physical vapor deposition developed for industrial exploitations generates pure and continuous thin layers of matter. It was successfully applied to the scarce examples of neutral and thermally stable SCO molecules [83] including Fe(phen)2(NCS)2. Original methods, like spray drying [84], self-assembling to form vesicular nanospheres [85], or using an easily processable block copolymer [86], were recently reported. The grafting of SCO molecules on pre-existing nanoparticles (SiO2 or Au) has led to hybrid systems with SCO-active interfaces [87]. The charge transport measurements of a device based on a gold nanoparticle array have shown an electrical response corresponding to the thermal SCO of the molecules located at the interfaces [88]. A complete overview of this subject opening toward nanostructured objects, nanocomposites, and applicative developments is given by L. Catala and L. Salmon in a specific chapter [89].
2 Recent results and perspectives
2.1 Usual coordinances
2.1.1 Ferrous compounds
The majority of mononuclear iron SCO materials are based on Fe(II) compounds with an N6 coordination sphere. For these materials, there is a constant development of new ligands and a detailed characterization of the corresponding iron complexes with the aim to understand and improve the SCO parameters, to increase the stability of the complexes for applications or to add new functionalities [90]. Some selected examples are given in Fig. 2. There is a continuous search for above RT SCO materials, SCO complexes with highly cooperative ST, or the combination of both (RT bistability) [91]. High-pressure structural investigations were used by Shepherd et al. [92] to get insight into the microscopic origin of cooperative interactions of the molecular SCO material [Fe(dpp)2(NCS)2]py. The high cooperativity of the SCO goes along with a large negative thermal expansion and a negative linear compression that are produced by a scissor-like geometrical mechanism of the complex. New examples for ferrous complexes with wide thermal hysteresis loops were reported that highlight the importance of hydrogen bond networks for the mediation of cooperative effects, for example, by Bonnet and co-workers ([Fe(bbpya)(NCS)2]) [93] or Abherve et al. [94] In few cases, the scan rate used for the magnetic measurements influences the width and temperature region, where thermal hysteresis loops appear [95]. A recent example of scan rate–dependent bistability due to different structural changes for the mononuclear Fe(II) complex [Fe(HLn-Pr)3]Cl(PF6) is reported by Fujinami et al. [96]. Another beautiful work was done by Seedyuk et al. [97] on complexes with short alkyl chains. The impact of different solvatomorphs on the spin state of the metal center and the SCO parameters is of special interest for applications in the field of sensing. Several new examples were published in the last few years [98], including one on a reversible single crystal to single crystal solvent exchange that influences the intermolecular cooperativity [99]. Luo et al. [100] synthesized the trisdiimine complex [Fe(H2Bpz2)2(bipy-NH2)] with an amino side group where they showed that protonation reversibly changes the properties of the complex from high spin to SCO at ambient temperature.

New ligands for the synthesis of Fe(II) SCO complexes.
Tridentate ligands as, for example, terpyridine analog pyridyl-phenanthrolines investigated by the Petzold et al. [101] yield very stable (solid, solution, and air) and durable complexes with tunable transition temperatures. Halcrow and co-workers synthesized a series of tridentate ligands to show that it is possible to control the spin state of the metal center via the ligand geometry [102], its chirality [103], and also through a control of the phase transition behavior [104]. Investigations in solution showed that the SCO parameters are anion and solvent dependent [105]. For a better understanding and control of the SCO parameters of trisdiimine complexes, Shatruk et al. [17] proposed a very simple and straightforward approach to predict the spin state of such complexes. Examples for Fe(II) SCO complexes with hexadentate ligands based on Schiff bases were investigated by Kulmaczewski et al. [106] and Heider et al. [107].
Next, to design new ligands with optimized properties, known systems are used to test their potential for applications. Tridentate ligands with a bpp core were functionalized in the outer periphery for surface deposition by Kuppusamy et al. [108]. SCO active complexes were deposited on the surface, for example, as thin film to measure the volume change upon ST with a microcantilever [109]. Zhao et al. [110] encapsulated mononuclear SCO complexes in a closed nanoconfinement in NH2-MIL-101(Al) for solvent-triggered spin-state switching.
2.1.2 Ferric compounds
An increasing number of works are dedicated to ferric SCO materials, which in their vast majority, incorporate Schiff base ligands as displayed in Fig. 3. Chemically stable and magnetically switchable ionic liquids have been elaborated by designing an L1 ligand, which allows the [Fe(L1)]((CN)2N)3·3H2O compound melting point to be properly shifted [111]. Amphiphilic Schiff base analogs exhibit a gradual spin-state switching associated with a transition between a solid and a possibly ordered liquid phase manifested by a hysteresis loop close to RT [112].
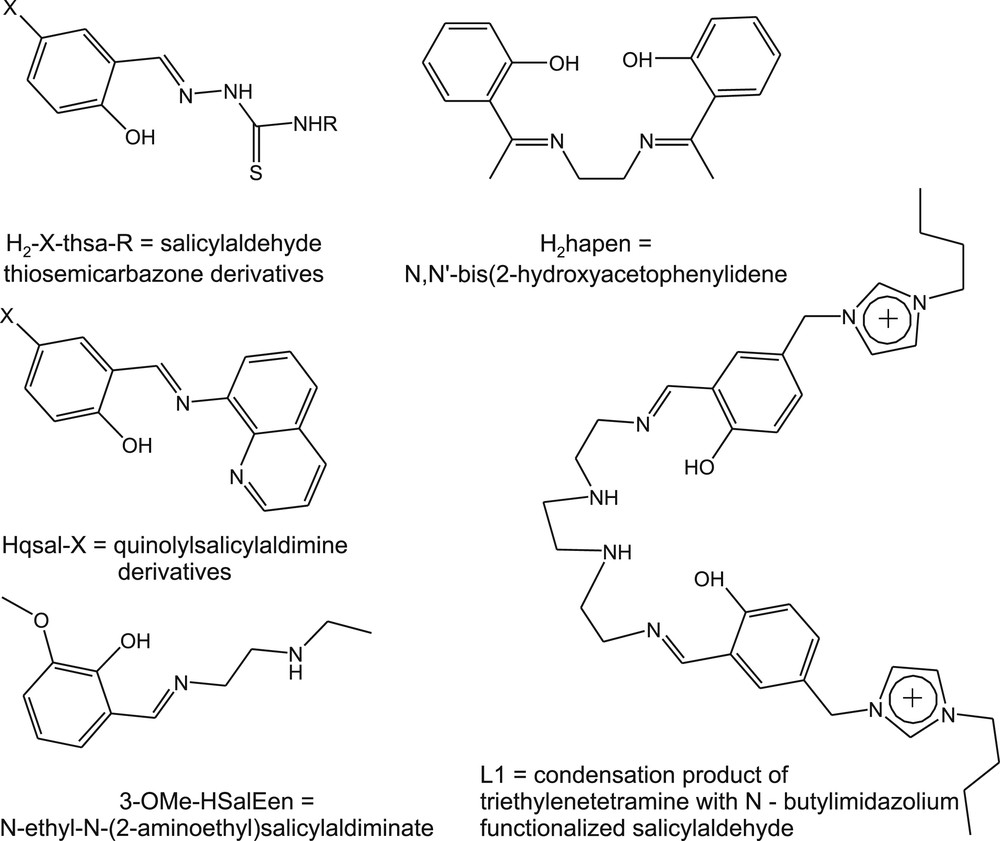
Ligands for the synthesis of Fe(III) SCO complexes.
Martinho et al. [113] investigated how the substitution of the phenolate ring of the 3-OMe-SalEen ligand changes the metal-centered reduction and the optical spectra in relation to the SCO properties of the [FeIIIN4O2] complexes at solid state. Solid state investigations of Fe(III) compounds have provided new examples of cooperative, stepwise, and hysteretic [114] behaviors. A thermosalient effect has been thoroughly studied with a new ST compound [Fe(5-Br-salEen)2][ClO4] showing an asymmetric hysteresis close to RT. This effect discernible in spectacular crystal jump, deformation, or fragmentation was rationalized in terms of mechanical stress accompanying the isostructural phase transition with a very anisotropic change in the lattice unit cell that was coupled with the ST [115]. The neutral Fe(III) complex with 5-Br-thsa-Me ligands exhibits a multistep magnetic hysteresis that takes place through intermediate re-entrant crystallographic phase transitions with symmetry breaking and spin-state ordering [116]. A neutral but solvated analog is another remarkable multistep system. Again a two-step symmetry breaking and a resonance-assisted hydrogen bonding involving the thiourea part of the molecule were observed, which played an active role in the cooperativity and the multistep ST [117]. The multifunctional character of this class of neutral [Fe(H-5-Cl-thsa-Me)(5-Cl-thsa-Me)]·H2O ST compounds was stressed from the observation of dielectric anomalies associated with the electronic and structural features and the photomagnetism based on LIESST [118].
2.2 Unusual coordinances (SCO without configurational relaxation), symmetry or charge
2.2.1 Compounds with unusual coordinances
Apart from the N6 coordination sphere, the N4O2 coordination sphere is commonly used for the synthesis of SCO complexes. Ortega-Villar et al. [119] developed a new family of Fe(III) SCO complexes with a singular N5O coordination sphere incorporating strongly basic anionic alkoxide ligands. Although tridentate Schiff base N2O ligands are mostly relevant for the large family of SCO active Fe(III) compounds [8], there are also a small number of Fe(II) compounds known with SCO at RT 91c or above RT [120]. Schiff base–like N2O2 coordinating ligands in combination with different monodentate N-heterocycles as ligands are used by the Weber group for the synthesis of SCO complexes [13]. These ligands are continuously developed further through modifications in the outer periphery to increase cooperativity by optimizing intermolecular interactions through extended π-system [121], introduction of OH groups [122] or alkyl side chains to control the self-assembly [123]. A rare example for an SCO active mononuclear complex with an N4S2 coordination sphere provided by the tetradentate ligand S,S′-bis(2-pyridylmethyl)-1,2-thioethane and two NCE (E = S, Se, BH3) coligands is reported by Arroyave et al. [124]. There are few SCO active {FeNO}7 iron nitrosyl complexes that are either penta- or hexacoordinated [12,15]. A highly stable nonheme nitrosyl Fe(II) SCO complex with an S = 1/2 ↔ S = 3/2 spin-state change above RT (370 K) was reported in 2016 by Pineiro-Lopez et al. The strong antiferromagnetic coupling between the NO radical (S = 1/2) and the ferrous S = 0 ↔ S = 2 center is the reason for the unusual spin state for this six-coordinated Fe(II) complexes [125]. Unusual coordination number associated with SCO is 7 or 4. In the first case, coordination number is 7 in the high-spin state and 6 in the low-spin state [53]. A detailed structural analysis on a series of these complexes by Wang et al. [126] now revealed synergetic effects between SCO and metal–ligand bond break. Scepaniak et al. [9] published the first example for a four-coordinated ferrous SCO complex in 2011. In a follow-up study, they showed that this complex is photoactive at less than 20 K [127]. In the photoinduced HS state, a slow relaxation of the magnetization is observed. Furthermore, it is possible to modulate the SCO properties in a series of related complexes through steric and electronic effects [128].
2.2.2 Heteroleptic compounds
Within the large library of SCO octahedral molecules incorporating ligands of different nature, that is, the heteroleptic compounds, the limited subset formed with ligands of a given denticity represents a nontrivial synthetic case in view of the chemical lability characterizing the iron chemistry. Recently, several interesting Fe(II) or Fe(III) compounds formed with tridentate or bidentate chelating ligands were reported. Phonsri et al. [129] focused on a series of polar SCO Fe(III) compounds in which the combination of H2-thsa and H-qsal ligands affords electrically neutral complexes directly isolated in the form of single crystals. When desolvated, some of them retain their structural integrity over different degrees of solvation and reabsorb solvent molecules in their porosity. SCO and hysteretic behaviors [130] particularize these Schiff-base compounds. Heteroleptic Fe(II) complexes are of high importance for subsequent functionalization, making the integration of the molecules in devices possible while preserving their switchable character. The stability of the LS bis-terpyridine Fe(II) complexes in solution has been exploited for preparing by a statistical approach, a set of analogs incorporating a push–pull dipolar ligand and an anchoring ligand. The molecular design has allowed the observation of a single-molecule spin switching that was triggered by the distortion of the coordination sphere under the effect of an applied electric field [131]. Phan et al. [132] succeeded with the well-known combination of polyimine ligands of different denticities and sequential syntheses in the isolation of compounds showing SCO, bistability, and photomagnetic behaviors. Aromi and co-workers investigated the propensity of trisimine ligands to form by mixing heteroleptic Fe(II) complexes in solution and at solid state. Remarkably, one of the nonporous materials exhibits a vapochromism due to an exchange of the solvent molecule taking place through a crystal-to-crystal reversible transformation associated with the spin-state switching of the metal ion [133]. Mixed ligands six-coordinate Fe(II) complexes are also targeted for applications in solar energy conversion [134], a strategic topic that requires abundant, inexpensive, and efficient photosentizers. Recent developments based on the engineering of the metal–ligand interaction with strongly donating N-heterocyclic carbene ligands have significantly improved the 3MLCT (metal-to-ligand charge transfer) lifetime with respect to the polypyridyl ligands [135]. Ni and co-workers report on an anion-dependent spin-state switching observed with Fe(II) diimine complexes at RT that may be relevant for the chemical sensing in solution. The preparation of mixed ligand complexes was achieved by using the sequential substitution of ligand in a precursor and the selective properties of solubility of reactants [136].
2.3 Multiple functionalities
2.3.1 Luminescence
The combination of SCO with luminescence would significantly broaden the application potential of SCO complexes into the direction of biosensors for nanothermometry [137] or fluorescence microscopy [138], provided a change in the intensity or wavelength of the emitting radiation upon the spin-state change can be realized. Two different strategies were used so far to realize such systems. Either SCO complexes were combined with luminescent materials in a composite material or the fluorophore is covalently bound to the SCO complex. In 2008, Matsuda and co-workers reported the fabrication of a temperature-dependent electroluminescent device based on a thin film of the SCO complex [Fe(dpp)2](BF4)2 doped with chlorophyll a (dpp = 2,6-di(pyrazol-1-yl)pyridine) [139]. It was demonstrated that the thin film chlorophyll a:[Fe(dpp)2](BF4)2 in its HS state is electroluminescent, but not in the LS state. When the doping material was changed to Nile Red (9-diethylamino-5-benzo[α]phenoxazinone), then the thin film was always electroluminescent regardless of the spin state. The authors concluded that the on/off switching is because of a change in the molecular orbital level of the complex [Fe(dpp)2](BF4)2 upon ST. Another example of a mononuclear system was reported by Cook et al. [140]. Different solid solutions of the SCO complex [Fe(dpp)2](BF4)2 and the phosphorescent complex [Ru(terpy)(terpy*)](BF4)2 were prepared by cocrystallization of both complexes. After demonstrating that only cocrystals were obtained, the magnetic properties were investigated. The cocrystals all exhibit SCO; however, the authors could not observe any emission upon irradiation in the solid state, regardless of the spin state of the Fe(II) complex.
The first example to couple luminescence and SCO through a covalent bond is from the Fe-triazole family of compounds. Garcia et al. reported the synthesis of a dinuclear Fe(II) complex where the triazoles have a salicylidene fluorescing group as substituent, presenting an abrupt ST at 150 K. The dinuclear complex exhibits one emission at λ = 394 nm in the LS state; however, in the HS state the dinuclear features two emission peaks: one is the emission of the LS state shifted to 414 nm, and another a new peak at 510 nm. In addition, the evolution of the intensity of the emission features with temperature is irregular in the region of the ST [141]. There are also examples where a fluorophore was attached to an SCO complex; however, no coupling or correlation between the spin state and the emission properties was observed [142].
2.3.2 Chirality
Chiral switchable SCO complexes offer interesting possibilities as elements in molecular and photonic devices due to alternative read-out possibilities [143]. Furthermore, the question arises, if chirality affects the nature of SCO, as this phenomenon is known to be influenced by smallest subfactors. In principle, for homoleptic complexes with three bidentate ligands or heteroleptic complexes with two bidentate and two monodentate ligands in cis position Δ and Λ enantiomorphs can be obtained. Often both enantiomorphs are involved in the crystal and a racemic compound is obtained, as, for example, shown by Brewer et al. [144] for an Fe(II) complex with a Schiff base condensate of 5-methylpyrazole-3-carboxaldehyde and tris(2-aminoethyl)amine as ligand. The first homochiral crystals of SCO complexes build through self-assembly were synthesized by Matsumoto and co-workers [145]. Bartual-Murgui et al. [146] scrutinized the subtle relationship between the homo- and heterochiral crystal packing, the polymorphism, and the SCO features. Liu et al. report the synthesis of a chiral SCO metal–organic framework through spontaneous resolution. The triply interpenetrated coordination network with quartz-like chiral topology shows a two-step SCO with a plateau at RT [147]. A further synthesis strategy was used by Ren et al., who report the synthesis of chiral tetrahedral Fe(II) cages with SCO close to RT [148]. Self-assembly of chiral subcomponents yielded enantiomerically pure SCO cages. The use of chiral ligands is another possibility for the synthesis of chiral SCO complexes [149]. In a very systematic approach, Sekimoto et al. [150] used the chiral (R and S) and racemic (rac) samples of a ligand to investigate the impact on the crystal packing and the SCO properties. With the chiral ligands the complexes crystallize as Δ and Λ enantiomers and have identical SCO properties, whereas for the racemic complex T1/2 is shifted to lower temperatures.
2.3.3 Photoresponsive switchable systems
New functionalized molecular materials including a photoresponsive organic moiety have been reported [44]. Tuczek et al. described a series of Fe(II) complexes formed with 4- or 5-phenylazo-2,2′-bipyridine ligands that exhibit a diversity of SCO behaviors (continuous, stepwise, hysteretic, or incomplete processes) in the solid state [151]. The study of the LD-LISC process is not presented but the spin-state switching occurs via a metal-centered photoexcitation (LIESST) at cryogenic temperature. In the case of Schiff base Fe(III) complexes dispersed in solution, it has been established that in contrary to a first report [152] the reversible isomerization of monodentate ligands does not allow a photomagnetic response to be detected as a consequence of the weakness of the ligand field strength modulation and the chemical reactivity of these ferric complexes [153].
The styryl or diazobenzene functions are characterized by optically reversible transformations in solution. When irradiated in confined media (crystalline solids), the switching may proceed via a one-way mechanism [45a] associated with an MLCT excitation [44]. In contrast to the Z/E isomerization, the cyclization of diarylethene is a volume-preserving process compatible with a crystalline photochromism that has a strong potential for the development of LD-LISC materials. Nihei et al. [154] and Khusniyarov [155] investigated a heteroleptic Fe(II) SCO complex with a diarylethene-based chelating ligand. In solution, the conversion with light of the organic moiety reversibly shifts the low temperature spin-equilibrium curve of the complex in one case, whereas it gives rise to a photomagnetic response detected at RT in the other case. The reversible spin-state switching of the solid resulting from the photoisomerization of the ligand was demonstrated at RT with a combination of surface-sensitive techniques [156]. These exciting results open a route toward molecular devices whose spin-dependent functionalities may be reversibly switched with light at ambient temperature and that at the nanoscale and ultimately the molecular scale. The LD-LISC phenomenon involves two localized processes—the metal ion SCO and the ligand photoisomerization—taking place via a structural rearrangement. Quantum chemical methods were applied at the molecular level for the determination of the ligand field strength and the ground state of the complexes [157]. These investigations are important for the chemical design of efficient systems, the understanding of the mechanism and the dynamics of the processes, and the implementation of LD-LISC-based molecular devices.
In a broader context, some recent works have addressed the question of how to slow down the kinetics of the spin-state interconversion in solution that is typically in the nanosecond timescale. Stock et al. [158] propose to use trigonal-prismatic Fe(II) complexes for which the coupling between breathing and torsional reaction coordinates results in a slow spin-state interconversion (millisecond timescale just below RT). Moreover, promising achievements also concern photochromic ligand–metal complexes, for instance, with valence tautomerism [159], metal carbonyl [160], or SCO polynuclear systems [161].
2.3.4 Redox compounds
The literature also distinguishes original specimen of SCO metal complexes with redox-active ligands. Scott et al. [162] focus on how ferrocene-appended ligands influence the capability of their d6 (or d7) metal ion complexes to show an SCO. Unfortunately, the redox properties have not allowed ferrocenium complexes of divalent metal ions to be isolated by electrolysis. The ferrocene-bearing triazole–pyridine ligands when coordinated with Fe(II) ions promote SCO and photomagnetic properties that may be attractive for studying synergetic effects with redox or nonlinear optical properties [163]. Schmitz et al. [164] have investigated the S = 1/2 to S = 3/2 SCO properties of an Fe(II) center interacting with a monoreduced diimine ligand, whereas an LS metal ion was found in the precursor. The chemical substitution of bis(pyridylimino)isoindoline chelates was exploited by Scheja et al. [165] for varying the redox properties of iron compounds from what they discovered, both SCO and valence tautomerism behaviors. Pentacoordinated iron complexes in which the radical bis(imino)pyridine ligand participates in the complex electronic structure were reported [166]. The low-spin (S = 0) to intermediate-spin (S = 1) transition was assigned to an SCO of the ferric ion antiferromagnetically coupled with the radical.
2.3.5 Amphiphilic/liquid crystalline materials
One possibility to realize multifunctionality is the combination of SCO with liquid crystalline (LC) properties [6,64,167,168]. The structural changes upon the spin-state change could trigger an LC phase transition or, alternatively, the phase transition could trigger the ST. Furthermore, the combination of LC and SCO properties offers the opportunity of an orientation of the complexes via the electrical field. In the case of amphiphilic systems, new strategies to process bulk SCO compounds into thin films also motivated such studies. Those could be obtained by techniques such as the Langmuir–Blodgett, spin coating, or drop casting.
One possibility to realize LC properties is the functionalization of known SCO complexes with long alkyl chains [168]. Bodenthin and co-workers showed that in the case of cationic SCO complexes the combination with amphiphilic counter ions leads to self-assembled systems, where the ST can by triggered by mechanical stress [169] or an LC phase transition [170]. The SCO properties of the system can be tuned by a variation in the ratio of metal ion to amphiphilic anion, which controls the self-assembled structures. In contrast to this the chain length does not significantly influence the ST temperature. The direct functionalization of the ligands of SCO complexes with long alkyl chains goes along with a significantly high synthetic effort. By this, the materials are stable in different media and much more flexible with regard to the application potential. The self-assembly and phase transition behavior of the complexes can then be investigated in the solid [167,171], on interfaces [172], and in solution [173,174]. There are several different systems based on Fe(II) [167,174,175] and Fe(III) [173,176,177] complexes. In most cases, the properties of the complexes were investigated in the solid. These investigations showed that the solid–liquid crystal, glass–liquid crystal, or gel–solution phase transitions can induce or modify the ST. Reasons for this are differences in the intermolecular contacts and the supramolecular arrangement for the different phases. However, especially for mononuclear complexes, investigations in solution are highly interesting because of the possibility to observe self-assembly. An interesting result from investigations on amphiphilic Fe(III) complexes in solution was obtained by Martinho et al. [177] An increase in the length of the alkyl chains did lead to higher cooperativity of the SCO in solution because of an improved self-assembly. Interestingly, such a behavior is not only observed in solution, but also in the solid state [173]. However, not all complexes bearing long alkyl chains have LC properties. For Fe(II) complexes with amphiphilic Schiff base–like ligands self-assembly behavior was observed but no LC properties [178]. The complexes crystallize in lipid layer–like structures. In agreement with the results from Gandolfi et al. [174] longer alkyl chains support the formation of lipid layers and by this lead to improved SCO properties [179]. In one case, an over 20 K wide thermal hysteresis loop is observed [27]. In Seredyuk and co-workers, an Fe(II) complex with short alkyl substituents was synthesized that exhibits two structurally different low-spin phases and one high-spin phase. Depending on the scan rate, two distinct well-separated strong cooperative STs are observed [180]. In a subsequent work, they showed that long-chain complexes display a more gradual SCO and crystal–liquid crystal phase transition [181]. Further optimization of the system showed that a phase transition in meltable complexes can be used to control the ST [182]. For this series, the transition temperature and hysteresis width do not rely on solid-state cooperative interactions but uses the coupling between phase and ST.
2.4 Coordinance change and spin-state switching in a few remarkable SCO inactive systems
One interesting potential application of SCO complexes in the field of sensing is as smart contrast agents (CAs). In the HS state, they reduce the longitudinal relaxation time T1 of surrounding solvent molecules whereas leaving it unchanged in the LS state. First works did focus on the temperature dependence. Janiak et al. [183] monitored the T1 shortening of D2O of a mononuclear Fe(II) triazole complex and Muller et al. [184] proposed polymeric Fe(II) triazoles as CA for hyperthermia. A useful strategy to realize a very large relaxivity gap (pronounced T1 shortage) is to generate a free coordination spot in the high-spin state where water molecules can be effectively exchanged [185]. This idea was investigated by Stavila et al. [186] who compared the relaxivities of structurally related iron complexes and found a large relaxivity difference between HS and LS states. Herges and co-workers used a reversible CISSS to design smart CAs based on Ni(II) complexes triggered by tissue properties different from temperature [187]. Coordination-induced switching effects on Fe(II) complexes were investigated in detail by Hasserodt et al. [188]. They demonstrated how irreversible coordination changes in a single molecule influence relaxation rates as a result of different spin states. In contrast to Gd(III) chelates that are never MRI silent due to outer-sphere relaxation [189], the diamagnetic LS state of Fe(II) complexes is completely MRI silent [190]. In 2017, Nowak et al. [191] reported a completely reversible spin-state switch of the naturally diamagnetic tris(bipyridine)iron(II) complex and the SCO complex bis(2,6-bis(1H-pyrazol-3-yl)pyridine)iron(II) by variation in the pH of solution and encapsulated in the supercages of zeolite. On the basis of the investigations in solution using 1H NMR spectroscopy, magnetic measurements, UV–vis spectroscopy, and molar relaxivity measurements, a proton-driven CISSS was identified as an underlying mechanism. At lower pH one of the nitrogen donor atoms of the N-heterocyclic ligand is protonated leading to a reversible FeN bond break. Because of the formation of a free coordination site, the presented systems are promising candidates for pH-responsive CAs in MRI.
3 Conclusions
There is an unbroken interest in mononuclear iron-based SCO complexes. Provided the necessary stability is ensured, they can be deposited on surfaces, encapsulated in porous materials or polymers, if necessary through the introduction of suitable anchoring groups. Through ligand design and/or the synthesis of heteroleptic complexes multiple functionalities can be easily reached. Although many of the concepts for the synthesis of nanoparticles rely on coordination polymers, there is still a wide spectrum of methods available to nanostructure mononuclear complexes. A big advantage of these systems is that remaining HS or LS fractions due to chain ends usually observed for polymeric systems do not exist. An ultimate goal, of course, is to address a few discrete molecules or a single molecule to control the properties at interfaces, to reach the highest possible data storage density, which can only be reached for molecular systems.