1 Introduction
Implication of silicate-based nanomaterials in electrochemistry has becoming a tremendous center of interest in the past two decades [1]. Several reviews or monographs are available, dealing with both the fundamentals and applications of electrodes modified with clays [2–7], zeolites [6–16], silica or silica-based materials [17–29]. Such a craze for the intersection between electrochemical science and the chemistry of these materials can be attributed to a combination of two complementary views. On the one hand, electrochemical techniques were applied to characterize the materials properties (redox activity of species incorporated into their porous structure by either ion exchange, adsorption or complexation, catalytic behavior, mass transport issues, …) [2,4,6,9,12,16,17,25,29]. On the other hand, and this constitutes probably the main driving force in the field [1], the attractive intrinsic features of these materials have been extensively exploited in electrochemistry via several advanced applications, including electroanalysis and sensors [5,7,11,14,18–21,26–28], biosensors [22,26], electrocatalysis [10,13,15], spectroelectrochemical devices [30,31], solid electrolytes and power sources [1,17,25]. Of special interest are the sol-gel derived materials and/or the organic–inorganic hybrids [32] due to the versatility of the sol-gel process to prepare a wide range of ceramic-based materials through the hydrolysis and condensation of metal alkoxides, alone or in the presence of one (or more) organosilane(s) precursor(s) to get organic–inorganic hybrids of class II (characterized by strong bonds between the organic and inorganic components [33]). These latter offer the advantage to combine in a single solid both the mechanical stability of an inorganic framework with the particular chemical reactivity of organofunctional groups strongly attached to the porous support.
Recently, at the junction between the fields of zeolite-modified electrodes and the electrochemistry involving silica-based materials, the ordered mesoporous silicas (MPS) were found to be appealing materials offering potential contributions to electrochemical science. Indeed, these ordered mesoporous solids discovered in 1992 [34,35], having regular, well-defined, and uniform channels varying typically from 20 to 100 Å (and even up to 300 Å), are displaying both the molecular sieving properties of zeolites (with much larger pores than the usual microporous aluminosilicates) and the chemical reactivity of the amorphous silica surface (e.g., they can be covalently grafted with organic groups [36], or their organic–inorganic hybrid forms can be prepared in one-step according to the sol-gel process in the presence of a structure-directing agent [37,38]). As mentioned at several places in this special issue and elsewhere [39–43], simple, versatile, and reliable methods are now available to obtain mesoporous molecular sieves with a wide range of structures, compositions and properties.
The present review intends to describe and illustrate the recently arisen intersection between electrochemistry and the chemistry of ordered mesoporous solids. It will focus only on silica-based materials and not on other mesoporous compounds, yet largely exploited for electrochemical purposes, as ordered porous carbon materials [44,45], mesoporous metal oxides (especially TiO2, among others [46,47]), electrically-conductive oxide aerogels [48–50], nanostructured metals [51,52], or other tailor-made nanomaterials [53]. In particular, emphasis will be given here to highlight the interest of ordered MPS-based materials for advanced applications in electrochemistry (preconcentration analysis and sensors, electrocatalysis, bioelectrochemistry, gas sensing) and, in turn, the application of electrochemical methods to characterize the materials properties (redox activity of immobilized electroactive probes, monitoring of mass transfer processes in organically-modified MPS).
2 Interest of ordered MPS in electrochemistry
The ordered MPS constitute a ‘novel’ class or porous materials that are commonly prepared by inorganic polymerization in the presence of a liquid-crystal-forming template (ionic or non-ionic molecular surfactant, block copolymer). These are amorphous solids characterized by cylindrical mesopores ranging from 20 to more than 100 Å in diameter, spatially organized into periodic arrays that often mimic the liquid crystalline phases exhibited by templates [34,35,54,55]. Removal of the hybrid mesophases (by calcination or extraction) generally affords stable mesoporous materials with extremely high surface area (up to 1400 m2 g–1), mesopore volume greater than 0.7 ml g–1 and narrow pore size distribution. Pure silica mesoporous molecular sieves exhibit three structure types: hexagonal (1D regular hexagonal packing of mesopore channels), cubic (3D bi-continuous system of pores), and lamellar (2D system of silica sheets interleaved by surfactant bilayers), as illustrated in Fig. 1.
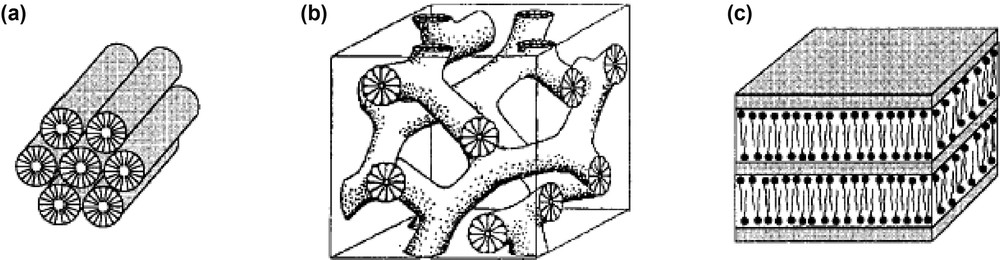
Three structure types observed for silica-surfactant mesophases: (a) hexagonal; (b) cubic bi-continuous; (c) lamellar. Reprinted with permission from N.K. Raman, M.T. Anderson, C.J. Brinker, Chem. Mater. 8 (1996) 1682. Copyright (1996) American Chemical Society.
Such inorganic solids displaying both high specific area and 3D structure made of highly open spaces interconnected to each other are attractive with respect to their use in connection to electrochemistry. Indeed, they provide a high density of reactive sites and their regular structure would impart high diffusion rates of target probes. This latter point is essential as most electrochemical transformations are diffusion-controlled. Another attractive feature is related to the versatility of the sol-gel process to prepare a wide variety of materials in ‘chimie douce’ conditions, which are compatible with the formation of, e.g. MPS films on solid substrates (electrodes) [56–59]. Also, the unique microstructural features of these mesoporous solids make them useful to be applied as mini-reactors for electron transfer reactions. For example, it has been demonstrated that 2,4,6-triphenylpyrylium ions incorporated in MCM-41 can be used as highly efficient electron shuttles (sensitizers) for the photoinduced conversion of cis-stilbene to trans-stilbene [60]. Mesoporous molecular wires have been also prepared in the form of encapsulated organic polymers [61–64], directly by in situ polymerization within the mesopores, and they were found to keep some electrical conductivity, yet greatly reduced compared to the same conducting polymer prepared in the bulk (see, e.g. polyaniline in MCM-41 [61]). These examples, together with other photoionization processes involving reactants immobilized inside MCM-41 or SBA-15 MPS [65–67], illustrate the possibility of charge transfer reactions in these mesostructured materials, opening the door for electrode-driven processes.
Of particular interest is the possibility to incorporate organic components within the MPS [36–38] because it brings additional physical and chemical properties to the materials, which might be readily exploited for new applications [39]. Derivatization of MPS can be achieved basically via two synthetic methods. First, attachment of functional moieties to the surface of mesopore channels can be made by grafting organosilane reactants on pre-fabricated mesoporous supports (post-synthesis modification) [36]. Second, organic–inorganic hybrids can be obtained in one-step by sol-gel chemistry via the co-condensation of a tetraalkoxysilane and one or more organoalkoxysilane(s) (“one-pot” synthesis) [37,38], this latter approach leading to uniformly distributed organic groups in the whole sample in comparison to the less uniform surface coverage arisen from the grafting process [39,68–70].
These MPS-based hybrids are of interest in electrochemistry for several reasons.
- • They are robust porous solids made of regular open spaces uniformly distributed in the form of a 3D structure containing a rather high number of organofunctional groups covalently attached to the mesopore walls; this would lead to great and fast accessibility for reactants to the active centers in the materials. This is especially important for preconcentration analysis (involving the accumulation of the target analyte prior to its voltammetric detection), for which the diffusion processes contribute to great extent to the rate-determining step [14,26,71]. A proper choice of the organofunctional groups may also help in improving the selectivity of the binding event (accumulation) by inducing preferential or specific recognition of the analyte.
- • The mesostructured materials have found numerous applications in catalysis [72–74]; once immobilized at an electrode surface they could act as a support for charge transfer mediators that are promising for electrocatalysis purposes. With this respect, the mediator could even be part of the hybrid material used as the electrode modifier, in the form of the organic component attached to the mesopore walls, which would enhance long-term stability to the resulting electrochemical device.
- • Sol-gel nano-bioencapsulation has proven the ability to couple the physico-chemical features of inorganic materials and organic–inorganic hybrids with the selective binding, catalytic, and biosynthetic functions of biomolecules [75,76]. More recently, some efforts have been directed to use mesoporous molecular sieves as new supports for the encapsulation of functional biomolecules (especially enzymes), demonstrating that they retain their structural integrity and biological activity [77]. This opens the door to the development of integrated systems combining molecular recognition, catalysis, and signal transduction, for applications in the biosensor fields.
Finally, one should keep in mind that, beside the derivatized mesoporous solids, a wide range of functionalized silica gels are readily available as high surface area materials at lower cost. It would be therefore necessary to determine with respect to a target application if a particular functionalization is going on to have beneficial effects due to the ordered mesoporous structure. This will be also discussed in this article, when appropriate.
3 Strategies to confine MPS at electrode surfaces
Contrary to the intrinsically conductive mesoporous carbon [44,45], metals [51,52], and metal oxides [48–50], or the mesoporous TiO2-based materials that might be electrically-conductive [46,47], most nanostructured silica-based materials are electronic insulators. Their use in connection to electrochemistry would therefore require a close contact to an electrode surface or a composite conductive matrix likely to act as an electron donor or receptor, as previously described for zeolite- or silica-modified electrodes [1,8,11,16,21,26]. Several strategies have been applied for that purpose. They are summarized in Table 1, including the nature of the mesoporous materials and the electroactive species involved in the redox process or the target analyte (when appropriate).
Preparation methods for electrochemical devices containing MPS-based materials
Mesoporous materialsa | Methods and electrode configurationsb | Electroactive speciesc | Target analytes | References |
A. Dispersion of as-synthesized materials into CPEs | ||||
MCM-41 | 1. 10% MCM-41 + 60% graphite + 30% Nujol | HgII, CuII | [78,79] | |
APS | 2. 10% APS + 60% graphite + 30% Nujol | CuII | [80] | |
MPS | 3. 10% MPS + 50% graphite + 40% paraffin wax | HgII | [81] | |
MCM-41 or SBA-15 grafted with aminopropyl or mercaptopropyl groups | 4. 10% material + 60% graphite + 30% paraffin wax | HgII | [82] | |
SH-SAMMS | 5. CP + 20% mesoporous materials | PbII, HgII | [85] | |
Ac-Phos-SAMMS | 6. CP + 20% mesoporous materials | CdII, CuII, PbII | [86] | |
Carnosine-functionalized MCM-41 | 7. 10% material + 60% graphite + 30% paraffin wax | CuII | [83] | |
Sulfonic acid-functionalized MCM-41 | 8. 10% MCM-41 + 50% graphite + 40% paraffin wax | Cu2+, Ru(NH3)63+ | [84] | |
MCM-41 grafted with aminopropyl groups (protonated) exchanged with PMo12 | 9. 1 g PMo12 + 3 g graphite + 0.3 g paraffin wax | [P(Mo3O10)4]3– | BrO3–, ClO3– | [87] |
B. Devices based on pressed materials | ||||
MCM-41 grafted with ED, DET, ETD or DES | 1. MCM-41–graphite mixture (1:1) pressed on Pt grid | CoII (complexed) | [91,92] | |
MCM-48 or, SBA-15 doped with cytochrome c | 2. Mesoporous solid–graphite mixture (1:1) pressed on Pt gauze | Cytochrome c | [93] | |
MCM-41 grafted with aminopropyl groups | 3. Pressed pellet: 0.2 g MCM-41 + 0.2 g graphite | Cu(phen)22+, Mn(phen)22+ | [94] | |
Al-MCM-41 or MCM-41 | 4. Pressed pellet: MCM-41 + SnO2 powder + 2 embedded Pt wires | H2, CO, CH4 (gaseous) | [95] | |
C. As-synthesized materials—polymer films | ||||
HMS, Al-HMS | 1. 100 μl of a mixture [5 mg HMS + 1 mg PS in 1 ml THF] coated on ITO | Ru(NH3)63+ (adsorbed or ion exchanged) | [97] | |
Pdn0-SBA-15 (Pd nanoparticles in SBA-15) | 2. [Pd2+-SBA-15 + PVC + DCE] coated on GC, followed by electrochemical reduction of Pd2+ into Pdn0 nanoparticles | n Pd2+/Pdn0 | CO | [98] |
HMS | 3. 2 μl of a mixture [HMS (3 g l–1)] + PVA (0.15%)] + 2 μl Hb (0.1 mM in 0.1 M PBS pH 7) coated on GC | Hb | H2O2, NO2– | [99] |
MCM-41 containing encapsulated polyacetylene | 4. MCM-41/PVA bilayer caated on GC (2 g l–1 MCM-41 in acetone + 1 drop PVA in acetone) | Polyacetylene | [100] | |
BP@PMO of MCM-41 type | 5. MCM-41/acrylic resin bilayer coated on GC (50 μl of 2 g l–1 BP@PMO in acetone + 1 drop 1% Paraloid B72 in acetone) | Bipyridinium units | H2Q | [101] |
D. As-synthesized materials–carbon–polymer composite films | ||||
MCM-41 | 1. 40 μl MCM-41-C-PS suspension [30 mg MCM-41 + 30 mg C + 10 mg PS + 0.3 ml THF + 0.3 ml dichloromethane] coated on GC | Fe(phen)32+, Fe(bpy)32+, Ru(bpy)32+, Ru(bpy)2Cl2+ | [102] | |
MCM-41 pre-treated with Me2SiCl2) | 2. 2 drops of MCM-41-C-PS suspension [13 mg MCM-41 + 13 mg C + 6 mg PS + 0.5 ml dichloromethane] coated on Pt | Ferrocenyl dendrimers (impregnated) | [103] | |
E. In situ synthesized mesoporous films on solid electrodes | ||||
MCM-41 type film | 1. Precursor mixture [TMOS:CTACl:H2O:HCl (1:0.13:6.5:0.003)] spin-coated on ITO (+ calcination at 350 °C for 5 h) | Ru(bpy)32+, Fe(bpy)32+, Os(bpy)32+, Fe(CN)63–, Ru(CN)63–, Mo(CN)84– | [105] | |
SH-FMS film | 2. Precursor mixture [TEOS:H2O:EtOH:HNO3:F127 (1:8:8:0.05:0.008)] spin-coated on Au electrode array (+ calcination at 350 °C for 8 min), followed by grafting MPTMS in refluxing toluene | Pb2+ | [106] | |
Cubic SBA-16 type film | 3. Precursor mixture [TEOS:H2O:EtOH:HCl: F127 (0.0041:15:40: 0.16:0.098)] dip-coated as a sandwich between n-Si/SiO2/Si3N4 and a gold electrode | NO (gaseous) | [107] | |
Periodic ordered mesophases film | 4. Precursor mixture [TEOS:H2O:EtOH:HCl:CTAB (1:5:20:0.004: 0.14)] dip-coated on Si or Al2O3 substrate with Au-IDE (F127, Brij-58 & Tween-80 were also used as templates instead of CTAB) | H2O (gaseous) Alcohol vapors | [108,111] | |
Alcohol vapors | [109,110] | |||
F. Other | ||||
MCM-41 or Al-MCM-41 | 1. Electropolymerization of pyrrole containing MCM-41 particles in suspension (3.0 g l–1) on Pt or ITO | Pyrrole, polypyrrole | [104] |
a Abbreviations: APS, aminopropyl-functionalized silica; MPS, mercaptopropyl-functionalized silica; SH-SAMMS, thiol self-assembled monolayer on MPS; Ac-Phos-SAMMS, carbamoylphosphonic acid (acetamide phosphonic acid) self-assembled monolayer on MPS; PMo12, 1:12-phosphomolybdic acid; ED, ethylenediamine; DET, diethylenetriamine; ETD, ethylenediaminetriacetate; DES, diethylenetriamine-salicylaldehyde (Schiff base ligand); HMS, hexagonal MPS; BP@PMO, 4,4′-bipyridinium functionalized periodic mesoporous organosilica; SH-FMS, thiol functionalized MPS.
b Abbreviations: CP, carbon paste; PMo12, 1:12-phosphomolybdic acid; HMS, hexagonal MPS; PS, polystyrene; THF, tetrahydrofuran; ITO, indium-doped tin oxide; PVC, polyvinyl chloride; DCE, dichloroethane; GC, glassy carbon; PVA, polyvinyl alcohol; Hb, hemoglobin; BP@PMO, 4,4′-bipyridinium functionalized periodic mesoporous organosilica; TMOS, tetramethoxysilane; CTACl, cetyltrimethylammonium chloride; TEOS, tetraethoxysilane; F127, copolymer triblock Pluronic F127; MPTMS, 3-mercaptopropyl-trimethoxysilane; CTAB, cetyltrimethylammonium bromide; Au-IDE, interdigitated gold electrodes.
c Abbreviations: phen, 1,10-phenanthroline; Hb, hemoglobin; bpy, 2,2′-bipyridyl.
A first approach, largely applied by our group [78–84] and others [85–87], consists in the incorporation of as-synthesized mesostructured silicas or organo-silicas into a carbon paste matrix. The physical dispersion of (bio)chemicals and (bio)materials into a ‘graphite + binder’ mixture was indeed often exploited to prepare (bio)chemically modified electrodes [88–90]. This constitutes a convenient way of modification, intrinsically easy, which offers the advantage to enable the use of “sophisticated” chemical compounds or “high-tech” materials (i.e., mesoporous solids) as modifiers. This is particularly interesting when attempting to use mesostructured organic–inorganic hybrids that require somewhat harsh experimental conditions to be prepared and template extraction before use, which might be not compatible with direct synthesis at an electrode surface. The main drawback of this simple strategy, common with what was reported for zeolite-modified carbon paste electrodes [11,14], is the difficulty to get homogeneous composition of the resulting composite paste (homogeneous distribution of solid particles over the whole paste volume). This composition should be as more uniform as possible to maintain a good level of reproducibility after mechanical renewal of the electrode surface between each measurement. Almost all the applications relevant to this type of electrode modified with MPS were in the field of electroanalysis.
Another related method is based on the physical mixing of materials with conducting particles (most often graphite [91–94], but also tin oxide [95]), which was then pressed either on a Pt electrode [91–93] or, alone, in the form of pellets [94,95]. Again, this procedure mimics that first developed for zeolite-modified electrodes [96] and, as being binder-free, it offers the possibility to operate in organic medium. It gains also advantage to be universal, enabling the use of every kind of as-synthesized solid, but in case of mesoporous materials, careful attention should be paid to the possible crushing of the open structure during compression.
MPS-based materials were also deposited onto the surface of solid electrodes. Contrarily to clay modified electrodes but similarly to zeolite-modified electrodes [1], the simple evaporation of suspensions of the mesoporous particles did not produce robust films. An organic polymer was thus added in the suspension in order to get films that adhere well to the conductive substrates and were robust enough for handling. Composite coatings were then obtained as mesoporous solid particles dispersed in polystyrene [97], polyvinyl chloride [98], or polyvinyl alcohol [99], deposited on either indium–tin oxide (ITO) or glassy carbon substrates. An alternative approach relies on a two-step procedure involving the deposition of a first layer of MPS by simple evaporation of a suspension containing the solid particles, which is followed by overcoating with a polymer film, leading to a bilayer configuration [100,101]. Addition of carbon particles in the polymer-silica composite was sometimes performed to improve the electrical conductivity of the thin film [102,103]. Finally, one should also mention the encapsulation of MCM-41 particles within a semi-conducting film obtained by electropolymerization of pyrrole [104].
Direct generation of mesostructured films on electrically-conductive surfaces [56–59], likely to act as electrodes, provides probably the most attractive way to get a homogeneous and uniform periodic ordered mesoporous coating on an electrode surface. This has been achieved, thanks to the versatility of the sol-gel process and the fluid character of the sol (making it compatible with film formation), by depositing the ‘precursor + surfactant’ sol by spin-coating [105,106] or dip-coating [107–111] onto the surface of solid electrodes where the mesostructures were obtained. As illustrated in Fig. 2A, uniform and featureless mesoporous films can be obtained [105], contrarily to what was obtained by simple evaporation of suspensions of the mesoporous particles overcoated with a polystyrene film (Fig. 2C). Two critical points are associated to this procedure: the difficulty to get mesopores perpendicularly oriented on the electrode surface (to ensure efficient mass transport of electroactive probes or analytes from the external solution to the electrode surface) and the extraction of the template without damage to the mesostructure (to afford the active centers accessibility). Template extraction has been performed by calcination in case of pure silica mesoporous films [105–111], leading to a small decrease in the film thickness and appearance of some cracks (Fig. 2B) most probably due to strain introduced by the shrinkage of the mesoporous framework during calcination [105]. This process is however prevented for organically-modified silica films for which solvent extraction should be applied or, alternatively, the functional groups could be incorporated within the film by post-synthesis grafting [106]. Careful examination of the film after template removal, by high resolution transmission electron microscopy (Fig. 3), reveals that well-ordered hexagonal mesostructures can be obtained [105]. In principle, mesoporous films of tailor-made composition, structure, and properties, could be obtained by appropriate choice of the experimental conditions, but the full control of these parameters remains a challenge. New synthetic directions for getting sol-gel materials on electrode surfaces, e.g. the electro-assisted generation of functionalized silica coatings self-assembled on gold [112], might contribute to novel emerging intersections between electrochemistry and materials science. Another critical development in the field of thin organically-modified silica films on solid substrates relies on the possibility to prepare such mesoporous coatings functionalized either with positively- or negatively-charged moieties, in one-step, by combining the co-condensation synthetic route with dip-coating or spin-coating processes [113,114].
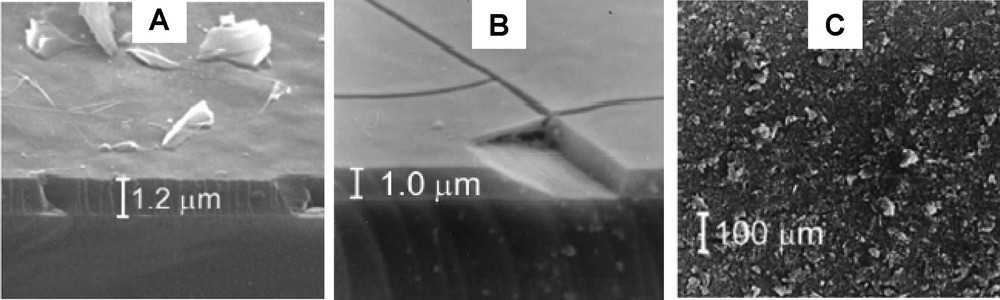
SEM micrographs of a film of MCM-41 obtained by spin-coating a mixture of CTACl (11% w/w) and TMOS (TMOS/CTACl molar ratio = 7.5) at 6000 rpm showing the edge of the film before (A) and after calcination (B). (C) Micrograph of an electrode coated with 200 μg of MCM-41 powder overcoated with 40 μg of polystyrene. Reprinted and adapted from Ref. [105].
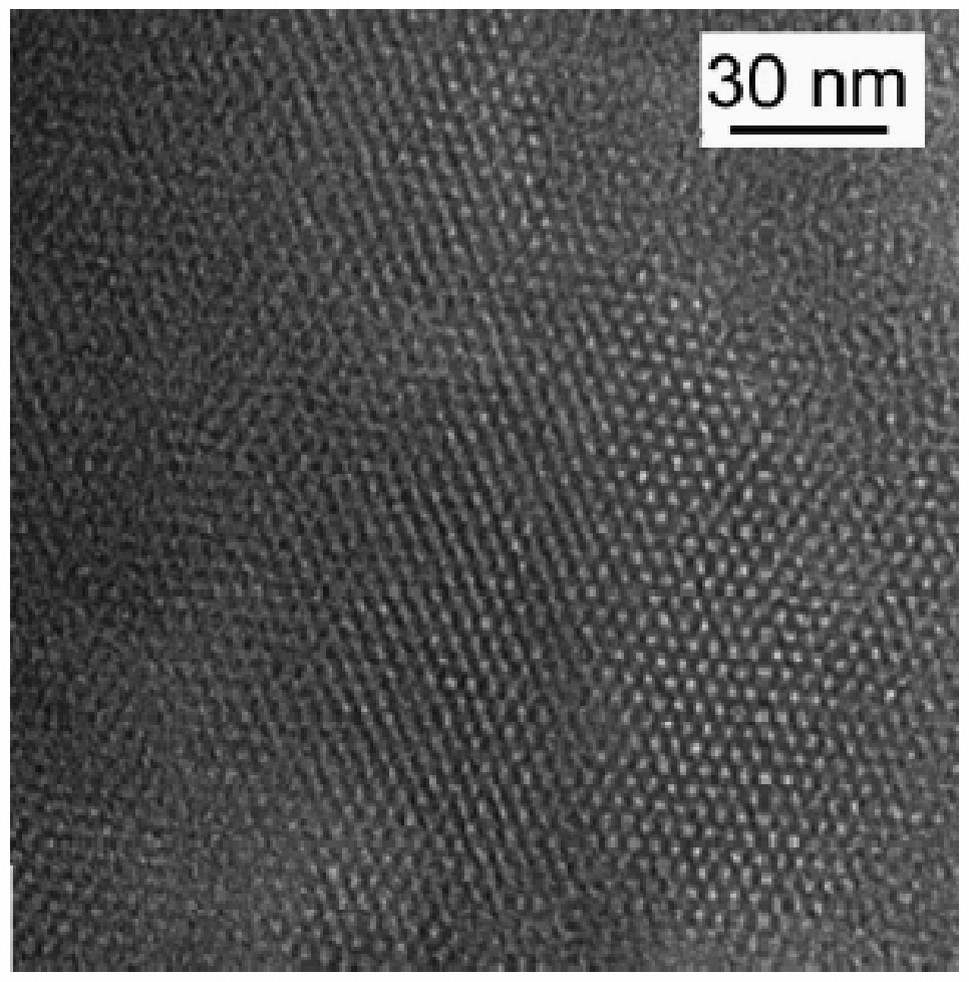
TEM micrograph of a flake taken from a calcinated MCM-41 spin-coated film prepared as in Fig. 2B. Reprinted from Ref. [105].
4 Voltammetric response of electroactive species in ordered MPS
Electroactive moieties can be introduced in MPS by simple impregnation (leading to adsorbed or entrapped species), via ion exchange (mainly in Al-containing MPS) or complexation (to organic ligands previously anchored to the mesopore walls), or by covalent attaching species in the mesopores.
The first report describing the implication of ordered MPS in electrochemistry was provided by Diaz et al. [91,92]. They described the preparation of a series of cobalt(II) complexes formed by exposing Co(II) solutions to several amine-derivatized MCM-41 particles. After pressing the materials in mixture with graphite particles onto a Pt grid, their electrochemical activity was probed by cyclic voltammetry (CV). In the presence of ethylenediamine (en) in solution (necessary to prevent irreversible reduction of Co(II) complexes), CV signals were indicative of a reversible redox couple at potential values close to those characteristics of the Co(en)32+ complex in aqueous medium. They were stable over extended period of time, even in conditions of multiple potential scanning cycles, suggesting the electrochemical response was indeed due to the cobalt complexes attached to the mesoporous support [92]. Similarly to what occurred for organometallic complexes physically encapsulated in microporous zeolites [115,116], only a small percentage of the total Co(II) complexes anchored to the mesoporous solid were electroactive. These species were those located at the outermost boundary of particles and/or at the entry of the mesopores because they were in closer contact to the conducting part of the electrode (graphite particle or Pt grid). Similar low intensity CV responses were obtained for copper- and manganese-phenanthroline complexes immobilized in MCM-41 particles, either as metal ion complexes simply incorporated by impregnation or by complexation to aminopropyl-grafted MCM-41 samples [94].
Much higher current responses can be obtained when forcing the electroactive species to move out of the insulating mesoporous support prior to performing the voltammetric detection. We have demonstrated this feature in several cases: the ammoniacal complexes of Cu(II) (or Hg(II)) immobilized within MCM-41 particles by ion/ligand exchange on the silanol groups (Eq. 1) [78], the chemisorbed Hg(II) species on the mesopore walls of MCM-41 (Eq. 2) [79], and Cu(II) species complexed to aminopropyl groups grafted in mesoporous MCM-41 particles (Eq. 3) [80].
(1)
(2)
(3)
All these equilibria are strongly pH-dependent and are expected to expel metal ions (Cu2+ or Hg2+) from the silica surface when decreasing pH. When these heavy metal-loaded MCM-41 particles were dispersed into carbon paste matrices and the resulting electrodes were immersed in acidic solutions, well-defined and intense voltammetric signals were observed. They correspond to the electrochemical activity of the metal ions expelled from the mesostructures at the electrode/solution interface. Most often, detection was achieved in the anodic stripping mode: metal ions were first reduced at a constant cathodic potential, into the corresponding metallic deposits, which were then re-oxidized by scanning potentials in the anodic direction. In this case, all the incorporated species were likely to be electroactive and the voltammetric signals were found to decrease by increasing the immersion time of the electrode in the electrolyte medium due to progressive leaching of Cu2+ or Hg2+ species in the solution. It is interesting to notice that the ordered mesoporous sorbents doped with these metal species gave rise to peak currents higher than those achieved with the corresponding amorphous solids (e.g., silica gels) [82], most probably because of less restricted mass transfer in case of ordered structures (see Section 5).
The Villemure group has provided two detailed studies describing (1) the voltammetric response of Ru(bpy)32+ electrostatically bound to the framework of mesoporous molecular sieves powder (HMS and Al-HMS) overcoated with polystyrene (PS) [97], and (2) the electrochemical activity of various cations (Ru(bpy)32+, Fe(bpy)32+, Os(bpy)32+) and anions (Fe(CN)63–, Ru(CN)63–, Mo(CN)84–) at spin-coated MCM-41 dense films on ITO (see corresponding micrographs on Figs. 2 and 3) [105]. Ru(bpy)32+ cations were incorporated in the mesoporous materials either by physisorption on the mesopore walls of HMS (0.07 meq g–1) or by ion exchange at the Al(III) sites in the framework of Al-HMS (0.47 meq g–1). A large fraction of both of them were electrochemically accessible because they retained enough mobility to diffuse out of the mesopores on the time scale of the voltammetric experiment, with peak potentials located at the same value as for Ru(bpy)32+ in solution, suggesting an extra-HMS electron transfer mechanism. Note that Jiang et al. [98] have reported the appearance of several additional peaks in the voltammetric curves of iron and ruthenium bipyridine and phenanthroline complexes adsorbed in MCM-41, accounting for possible influence of the silica surface on the electrochemical behavior of the incorporated cations. The apparent diffusion coefficient was larger for the weakly bound Ru(bpy)32+ species in the less densely filled HMS sample than for the numerous electrostatically bound cations in Al-HMS. The weakly bound Ru(bpy)32+ cations were easily removed from the HMS material by simple washing with water, giving rise to the suppression of the corresponding CV signals. When immersing the MPS modified electrodes (both HMS/PS coatings and MCM-41 dense films on ITO) in dilute Ru(bpy)32+ (or Fe(bpy)32+, or Os(bpy)32+) solutions, significant increase in peak currents was observed with time due to the accumulation of the electroactive cations in the mesoporous solids. This is exemplified on Fig. 4 for Ru(bpy)32+ at the HMS/PS-modified ITO electrode. The growth of CV peaks was faster for the composite HMS/PS coatings in comparison to the dense spin-coated films, most probably due to interparticle diffusion in the former case and also to the fact that mesopores are oriented parallel to the ITO substrate in the latter case. Even more interesting is the efficient blocking of the electrochemical activity of solution anions by the spin-coated films, while the porous HMS/PS coatings did not prevent these anions from reaching the electrode surface (Fig. 5). Such enhanced CV response for electroactive cations, associated to the completely blocked access of anions, was ascribed to the well-ordered mesopores (Fig. 3) in the dense spin-coated film (Fig. 2) made of pure silica (unmodified) [105]. When working with amorphous sol-gel films, such a charge selective behavior was only achieved in the presence of additional organic groups displaying a net charge (e.g., organically-modified silicate films containing either –NH3+ or –COO– groups covalently linked to the silica framework [117]).
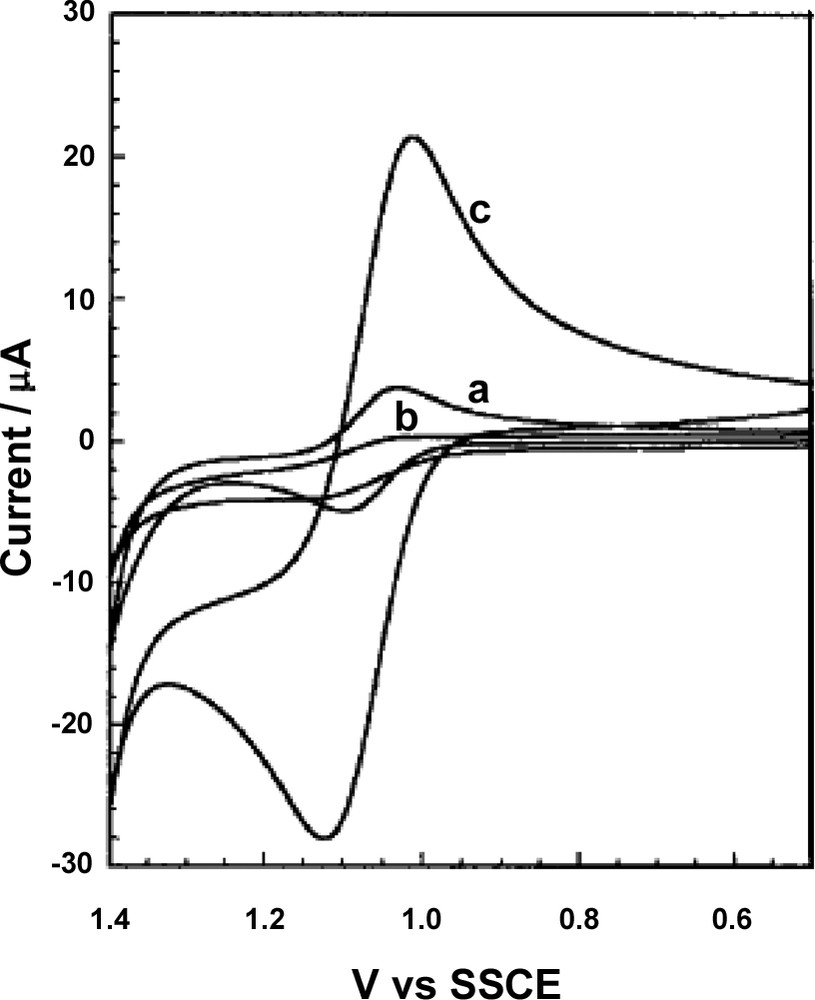
Cyclic voltammograms recorded at 50 mV s–1 in 0.05 M Na2SO4 electrolyte containing 0.05 mM Ru(bpy)32+: (a) bare ITO and HMS/polystyrene-modified electrode (b) 1 min and (c) 5 h after the film was placed in the solution. Reprinted with permission from Ref. [97].
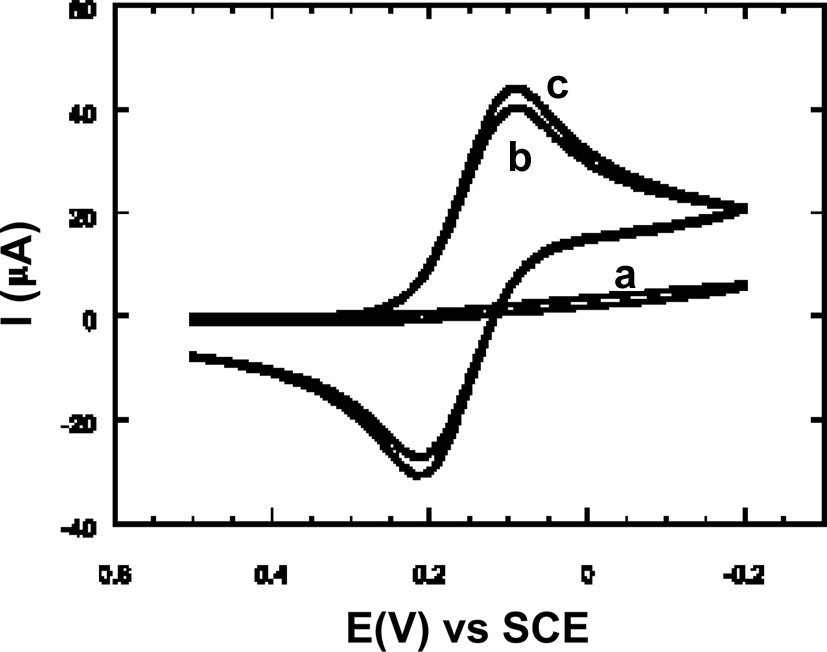
Cyclic voltammograms of (a) 1.0 μm spin-coated films 2 h after the film was placed in the solution, (b) MCM-41 polystyrene overcoated film and (c) bare ITO substrate (at 50 mV s–1 in 0.05 M potassium hydrogen phthalate containing 0.5 mM Fe(CN)63–). Reprinted from Ref. [105].
Another interesting work highlighting the attractiveness of MCM-41 in electrochemistry was performed by Diaz et al. [103] who have studied the voltammetric response of ferrocene-based dendritic guest molecules in highly ordered MPS. These molecules are poly(propyleneimine) dendrimers containing 4, 8, and 64 amidoferrocenyl moieties (1–3 in Fig. 6). Only the smaller molecules (1, 2) were readily incorporated in the regular channels of MCM-41 (nearly full occupancy by dendrimer 1 was obtained), the biggest one (3) leading to less effective inclusion. Durable immobilization was observed for molecules 1 and 2 due to hydrogen bonding interactions of the dendrimers (via their amide groups) with the surface silanol groups in the mesopores, while less stable immobilization was experienced with the bulkier compound 3. All these three dendrimer-containing composite materials gave rise to well-defined voltammetric signals, stable with time and upon continuous potential cycling. As dendrimers 1 and 2 cannot diffuse freely within the mesopore channels, the quite large electrochemical responses must be due to intra-MCM-41 electron transfer, by electron hopping, which is likely to occur between closely associated ferrocenyl moieties in the regular environment provided by the ordered mesopores. This constitutes the first example of electron transfer inside MPS, between guest molecules incorporated in the mesopores, which takes advantage of the high level of order to maintain the electroactive probes in sufficient proximity over extended length scale.
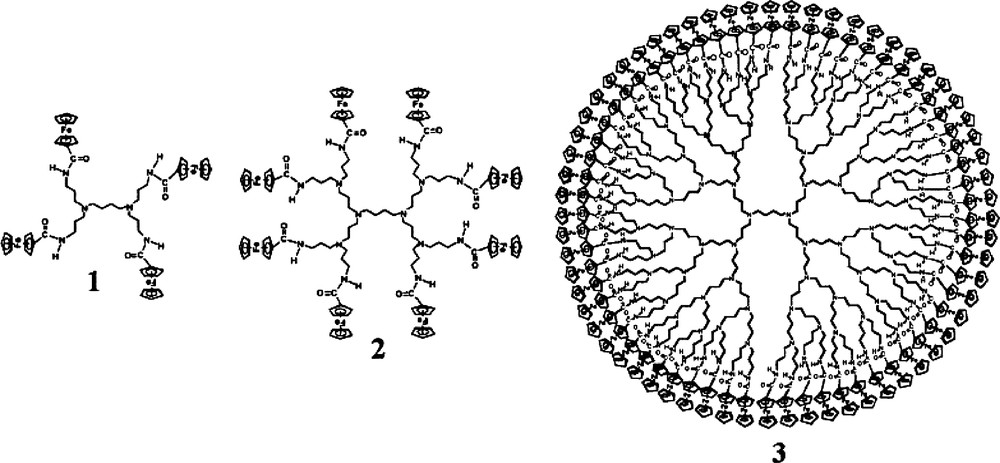
Poly(propyleneimine) dendrimers containing 4, 8 and 64 amidoferrocenyl moieties. Reprinted with permission from Ref. [103].
Other kind of electrochemically-induced charge transfer in MCM-41 materials involves the insertion of conducting polymers (polyacetylene [100] or polypyrrole [104]) in the mesopore channels. In these cases, of course, the electrochemical transformations do not require the intervention of electron hopping processes as the polymer chains provide electrical conductivity inside the porous materials.
5 Electrochemical monitoring of mass transfer processes in organically-modified MPS
It is now well-established that adsorbents designed from a mesostructure with spatially-ordered mesopore channels of uniform dimension are characterized by vastly improved access of guest species in comparison to the porous adsorbents prepared from materials with disordered pore networks (e.g., silica gels). This has been especially investigated for heavy metal ion binding to thiol-functionalized MPS, obtained either by post-grafting of preformed mesostructures [118–123] or by the one-step co-condensation method [124–129]. For instance, the total access of Hg(II) species to every binding sites (thiol groups) in the mesoporous adsorbents was demonstrated, providing the channel diameters were maintained in the mesopore range (>20 Å) upon functionalization [125,128]. This behavior is attractive for designing high capacity adsorbents, which are therefore promising to be used as electrode modifiers displaying a high number of binding groups.
Beside this definite advantage, the speed at which the guest species are reaching the active centers within the porous host is another important parameter affecting the performance of the material, especially in connection to electrochemical applications (operating most often under kinetic control). Thorough investigations on this aspect of the reactivity of functionalized MPS are not widespread [123,126,128,129]. After a brief survey of some important results obtained with classical approaches, two electrochemical methods developed in our group for probing mass transfer rates in mesoporous organic–inorganic hybrids will be described.
Protonation kinetics of various aminopropyl-grafted MPS (APS) samples displaying different structures (MCM-41, MCM-48, or SBA-15 types) and pore sizes (~30 and ~60 Å) have been studied by potentiometric monitoring of pH of APS suspensions in the presence of added protons (as HCl) at various H+/R-NH2 ratios [123]. They have been compared to the behavior of corresponding amorphous amine-functionalized silica gels [130]. Contrary to the Hg(II)–thiol system, less-than-complete reaction levels were achieved while attempting to protonate the ordered APS materials, suggesting that repulsive electrostatic interactions occurred in the regular confined medium. When operating with an amount of protons less than the APS capacity (<50%), very fast reaction was observed with the ordered solids (half-times of few seconds and even lower than 1 s for the large pore mesoporous materials), much faster than the protonation rates exhibited by the amine-functionalized silica gels. This illustrates the propensity for the uniform mesopores to induce fast mass transport processes. Note that protonation of amine groups by an acid HX also implies the diffusion of the counter anions, X–, in the material to maintain charge balance (Eq. 4), explaining why significant slowdown of the process was observed when increasing the size of this anion X– because of restricting motion of protons attempting to reach amine sites located deeply in the material [123].
(4)
By applying an appropriate diffusion model, and taking into account the particle size distribution, the apparent diffusion coefficients, Dapp, can be evaluated from fitting the kinetic data. Selected results are shown in Fig. 7, where Dapp values have been plotted against the state of reaction progress (Q/Q0 ratio, with Q = amount of reactant that has reached the active centers and Q0 = maximum amount of reactant that can reach the functional groups). As shown, protonation of ordered mesoporous APS samples is faster than for amorphous APS gels at low Q/Q0 ratio, while severe restrictions were observed when protonation was going on further. This limitation is thought to be due to electrostatic shielding effects, which were more pronounced with APS samples of smaller pore size (decrease of Dapp values at lower Q/Q0 ratios).
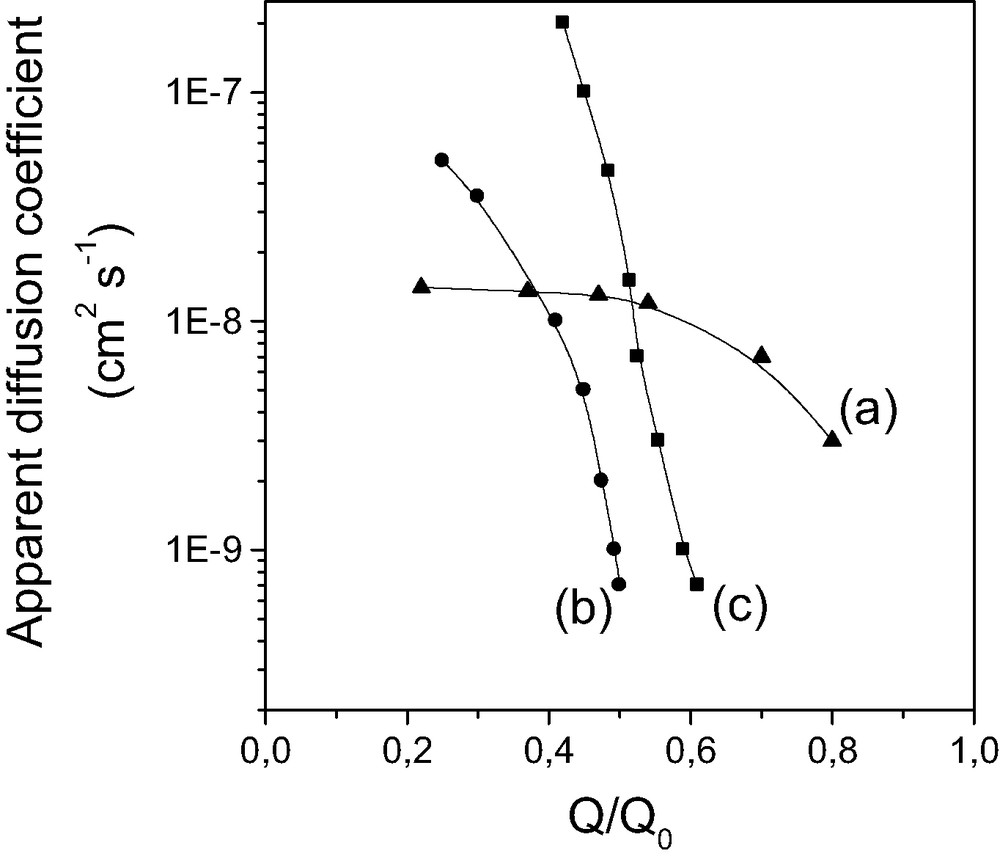
Variation of the apparent diffusion coefficient during protonation of various aminopropyl-grafted silica samples: (a) large pore (~6 nm) silica gel, (b) small pore (~3 nm) MCM-41, and (c) large pore (~6 nm) MCM-41, as a function of the extent of reaction (Q/Q0). Reprinted with permission from Ref. [123].
Bibby and Mercier [126] have studied the uptake kinetics of Hg(II) species by thiol-functionalized MPS microspheres (10–12 μm in diameter), displaying a MSU structure and a pore diameter of 2.5–3 nm, by the method of leftovers. Thiol-MSU particles were suspended in Hg(II)-containing solutions, solution-phase Hg(II) species were determined after selected periods of time, and the adsorbed amounts of Hg(II) were calculated by difference from the starting concentrations. The shrinking core model was applied to calculate the corresponding diffusion coefficients, which were in the range 10–11–10–10 cm2 s–1. This method of leftovers involving intermittent sampling operates quite well for these rather large particles with moderate pore size, but is on the wrong track in case of faster events (i.e., Hg(II) binding to smaller particles and/or more open structures). This is the reason why we have proposed an electrochemical method based on a rotating disk electrode to monitor in situ and continuously the consumption of Hg(II) in the suspension [123]. Typically, the electrode is complied to a cathodic potential value in conditions where steady-state currents are proportional to solution-phase Hg(II) species, the adsorbent particles are then added under stirring and Hg(II) binding is monitored via its consumption as a function of time. This enables to collect two data points every each second, which is compatible with fast uptake kinetics. We have applied this approach to the binding of Hg(II) species to a wide range of mercaptopropyl-grafted MPS and to the uptake of Cu(II) species by APS materials [123], and compared the results to the corresponding amorphous gels [130]. Unlikely to what was observed for the large thiol-MSU particles for which synergistic acceleration of the uptake rate occurred with increasing Hg(II) loading [126], Dapp values decreased slowly upon gradual filling (increasing Q/Q0 ratios, see Fig. 8) because less and less space was available for the ingress of metal species [123]. This decrease due to steric hindrance was much less than that induced by the repulsive electrostatic effects observed for protonation of APS materials (Fig. 7). Again, the ordered mesoporous solids exhibited faster diffusion processes (by about one order of magnitude) in comparison to amorphous gels of similar porosity (Fig. 8, comparison of curves ‘a’ and ‘c’ for Cu(II) binding to APS). One can also notice that faster diffusion was even ensured in the ordered mesostructure displaying smaller channel diameter than the average pore size of the amorphous gel (Fig. 8, comparison of curves ‘a’ and ‘b’).
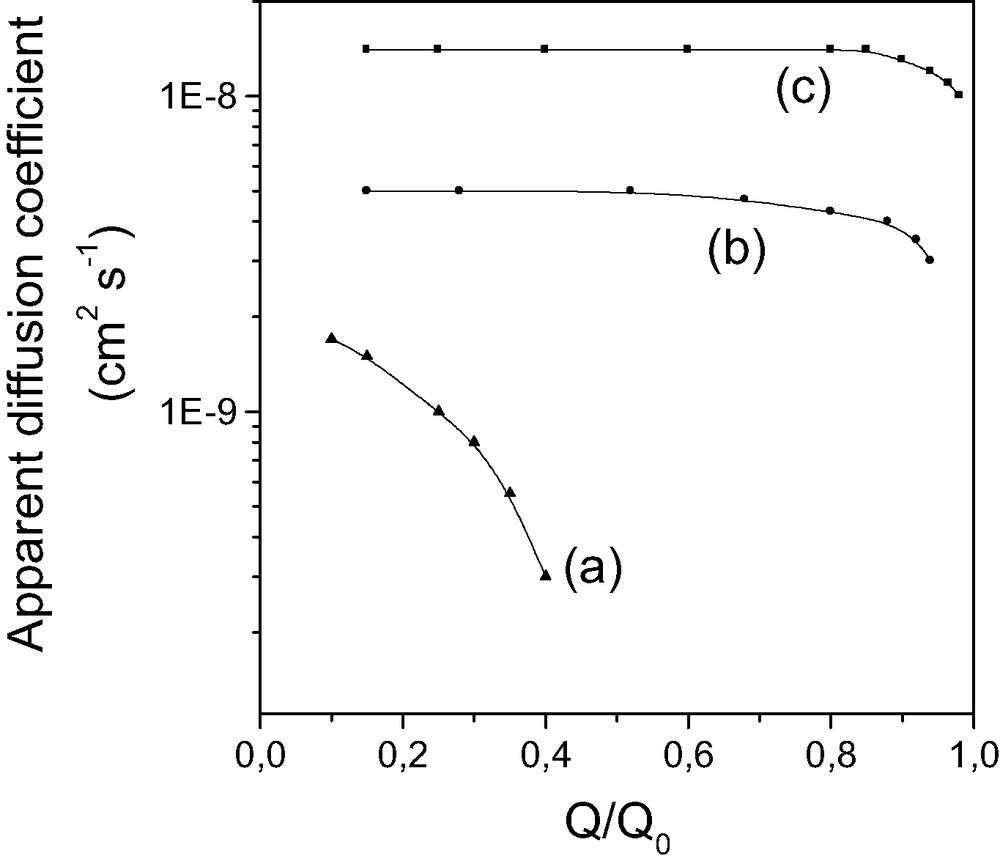
Variation of the apparent diffusion coefficient during the uptake of Cu(II) by various mercaptopropyl-grafted silica samples: (a) large pore (~6 nm) silica gel, (b) small pore (~3 nm) MCM-41, and (c) large pore (~6 nm) MCM-41, as a function of the extent of reaction (Q/Q0). Reprinted with permission from Ref. [123].
This electrochemical method was also applied to the analysis of Hg(II) diffusion in a wide range of MPS nanospheres obtained by the co-condensation route in the presence of a surfactant template [131] and featuring different functionalization levels (from 0.5 to 7 mmol SH per gram of material) [128]. This gave rise to several materials that can be classified into three categories (Fig. 9): (1) well-ordered structures with long-range hexagonal packing of mesopores at low thiol content, (2) wormhole-motif mesostructures with shorter range structural order at moderate functionalization levels, and (3) poorly ordered or amorphous solids containing huge amounts of thiol groups. Interestingly, varying the degree of functionalization enables one to highlight long-range versus short-range structural order/disorder in organically-modified MPS. Dapp values for Hg(II) species in MPS nanospheres were found to be rather low in well-ordered mesostructures, much better in materials ordered at shorter range in the form of wormhole-like arrangement of mesopores, and worse and worse in highly loaded, poorly ordered or amorphous, hydrophobic adsorbents (Fig. 10, curve ‘b’).
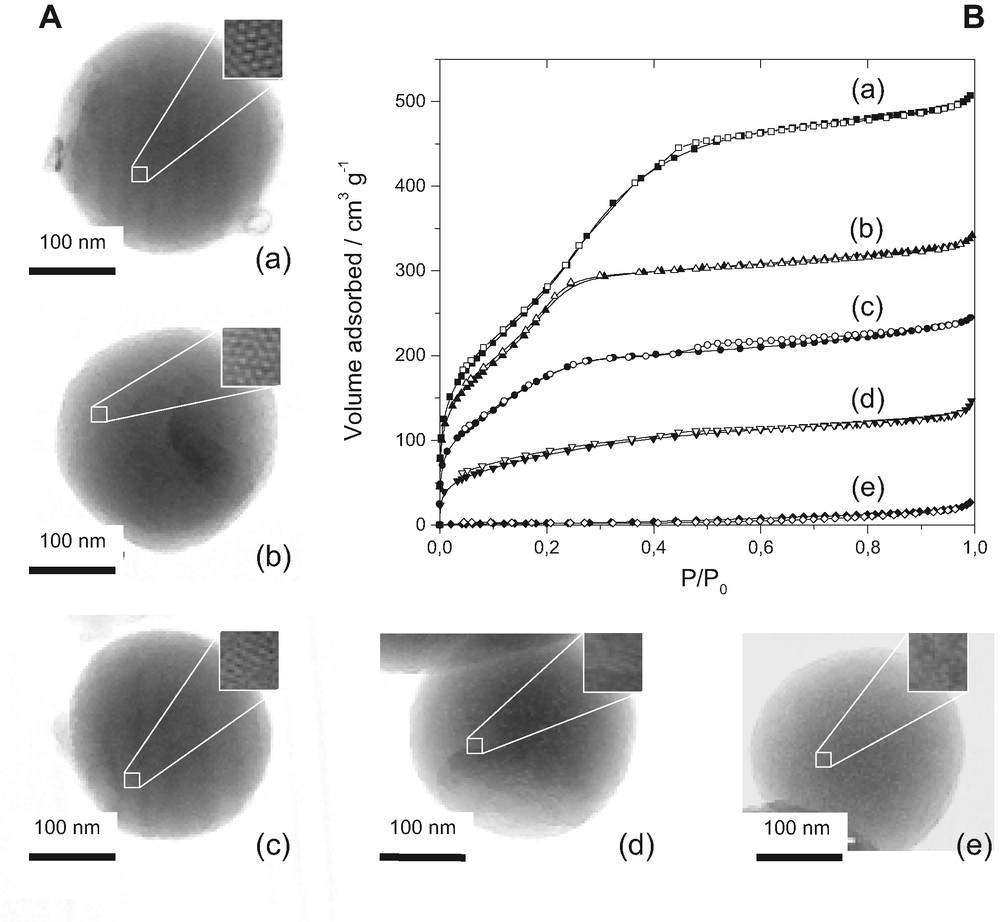
Transmission electron micrographs (A), powder X-ray diffraction patterns (B), and nitrogen adsorption(■)-desorption(□) isotherms (C), obtained for three cetyltrimethylammonium-assembled thiol-functionalized silica derivatives prepared by co-condensation of TEOS and MPTMS in various ratios in the starting sol: 10% MPTMS (10% SH), 20% MPTMS (20% SH), and 40% MPTMS (40% SH). Reprinted from Ref. [129].
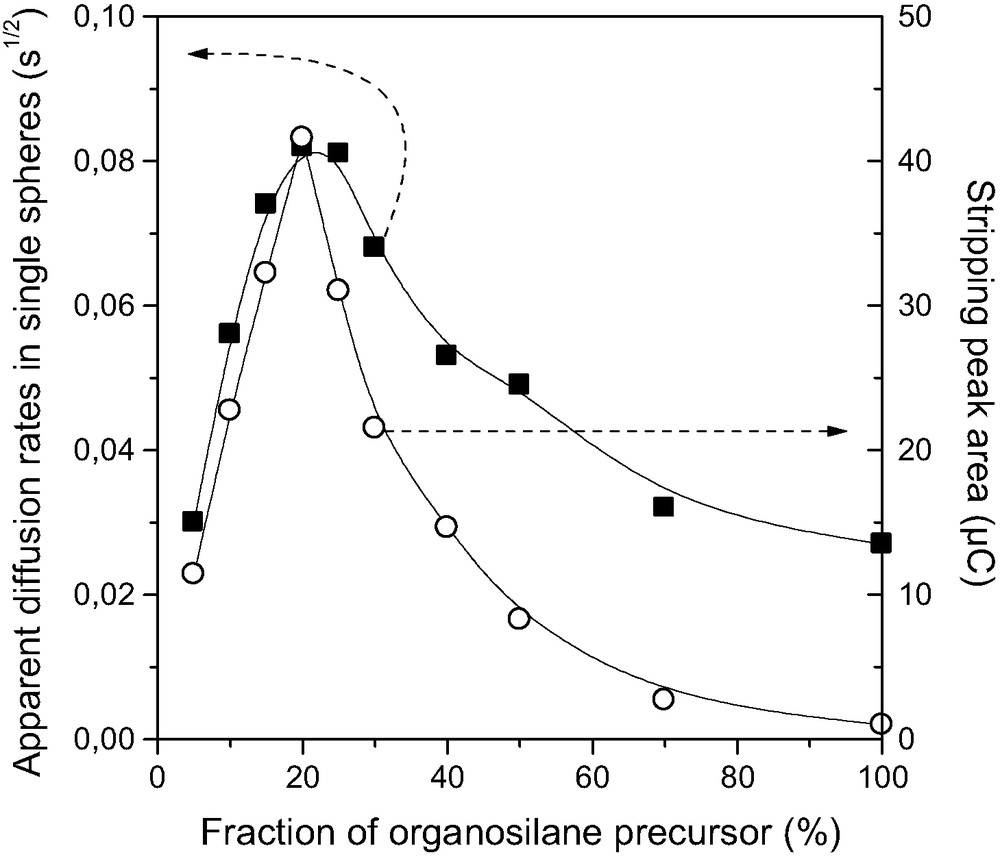
Electrochemical monitoring of the speed of HgII binding on thiol-functionalized silica spheres prepared, in the presence of CTAB, by co-condensation of TEOS and MPTMS in various ratios (materials noted MPS-n% (5 ≤ n ≤ 100)): variation of the voltammetric response (stripping peak area) of carbon paste electrodes modified with MPS-n% after 2 min accumulation from a 1 × 10–5 M HgII solution and detection by anodic stripping differential pulse voltammetry(○). Variation of the apparent diffusion rates of HgII in MPS spheres as a function of the relative molar ratio of MPTMS in the starting sol(■); data were estimated from kinetic curves (uptake of HgII as a function of time, from suspensions containing 20 mg of MPS-n% particles in 200 ml solution of HgII at an initial concentration selected to correspond to the maximal amount of accessible –SH groups), sampled at the early times of the experiments and expressed as the slope at the origin of the curve ‘fraction of HgII bound versus √t’. Reprinted from Ref. [129].
This series of mesoporous MPS materials was also exploited to develop a new electrochemical method for the qualitative or semi-quantitative analysis of mass transfer rates in organically-modified silica-based materials [129]. The method is based on the modification of carbon paste electrodes with the MPS particles and applying them in the preconcentration of Hg(II) from diluted solutions prior to voltammetric quantification, in conditions where the uptake process is diffusion-controlled. In this case, the recorded voltammetric signals constitute an indirect measurement of the rate at which Hg(II) species are reaching the thiol binding sites in the mesoporous solids. The validity of this approach is illustrated in Fig. 10, where the electrochemical response of the electrodes modified with MPS materials follows the same trend as that of Dapp values obtained according to the aforementioned rotating disk electrode technique. The modified electrode method operating in transient conditions (far below the equilibrium) is especially attractive when the classical approaches may undergo some limitations, e.g., in case of poor reaction yields (too low consumption of adsorbate from solution), in case of very fast uptake processes (too fast concentration fall, not measurable in solution), or even for adsorbents displaying rather low chemical stability in aqueous medium (long contact times forbidden). For instance, it allowed to characterize the rate of Cu(II) uptake by a wide range of mesoporous APS materials containing various amounts of amine groups, which cannot be studied by conventional methods, showing the same trend with respect to the functionalization level as that observed for Hg(II) on MPS [129].
All these examples demonstrate the interest of electrochemistry as a tool for characterizing the properties of mesoporous materials, especially in providing efficient methods to monitor mass transport issues in functionalized porous solids. Electrodes modified with MPS might be also useful to speciation studies, i.e, by determining whose chemical forms of a selected element are likely to interact preferably with the adsorbent in given experimental conditions. An example is available for the uptake of Hg(II) species by organically-modified silica gels, as a function of pH and chloride concentration, for which the resort to carbon paste electrodes containing these materials has led to pointing out distinct binding properties of the adsorbents with respect to Hg(II) speciation [132]. Beside the use of electrochemistry to characterize the materials properties, one can, in turn, exploit the intrinsic features of MPS-based adsorbents in several electrochemical applications, as described hereafter.
6 Preconcentration analysis
Chemically modified electrodes have been extensively used for trace analysis by exploiting the recognition and preconcentration properties of the modifier for a target analyte in order to accumulate it on the electrode surface prior to its detection by a suitable electrochemical technique [71,88–90]. The selectivity of the device is governed by the reactivity of the modifier towards the target analyte (characterized on thermodynamic basis) while the sensitivity of the detection step is related to the efficiency of the preconcentration event, which is most often limited by mass transfer processes (kinetic control). And this latter limitation is especially operating when using functionalized porous adsorbents as electrode modifiers, e.g., zeolites or silica-based materials, for preconcentration analysis [1,11,14,21,25,26]. From that point of view, on the basis of discussions made in Section 5, one could expect that the use of ordered mesoporous materials as electrode modifiers would result in high sensitivity of electrochemical devices applied in the ‘accumulation-voltammetric detection’ scheme.
Indeed, we have shown that tailoring the structure of organic–inorganic hybrids incorporated into carbon paste matrices enables to tune the sensitivity of the resulting modified electrodes. This was demonstrated for copper(II) sensing with electrodes modified by polysiloxane-immobilized amine ligands of various structure [80], and for the voltammetric detection of mercury(II) subsequent to open-circuit accumulation in mercaptopropyl-grafted amorphous silica gels and MCM-41 types materials [82] or thiol-functionalized silica samples displaying different level or ordering [81]. In agreement with kinetic observations (Section 5), higher sensitivity of the modified electrodes was usually achieved with the ordered mesoporous adsorbents in comparison to the corresponding functionalized silica gels. Typical illustration is given in Fig. 11 where one can clearly notice a 45-times increase in peak currents when passing from a thiol-functionalized silica gel of 6 nm pore size to a SBA-15 structure of the same pore aperture. Even more overwhelming, the sensitivity obtained with a MCM-41 material displaying channels twotimes smaller in diameter (3 nm) was higher (by a factor 8) than that achieved with the 6-nm pore size amorphous silica gel adsorbent [82]. Such advantage owing to the uniform mesostructure was also exhibited by pure (not functionalized) MPS-modified electrodes, as reported for sensing heavy metal species [78,79]. Of particular interest is the selective electrochemical detection of Hg(II) species after preconcentration at a MCM-41-modified carbon paste electrode, which is explained by the exclusive accumulation of Hg(OH)2 species via reaction with the surface silanol groups on the mesopore channels (Eq. 2), which is not affected by the presence of other metal ions in the pH range 4–7 [79].
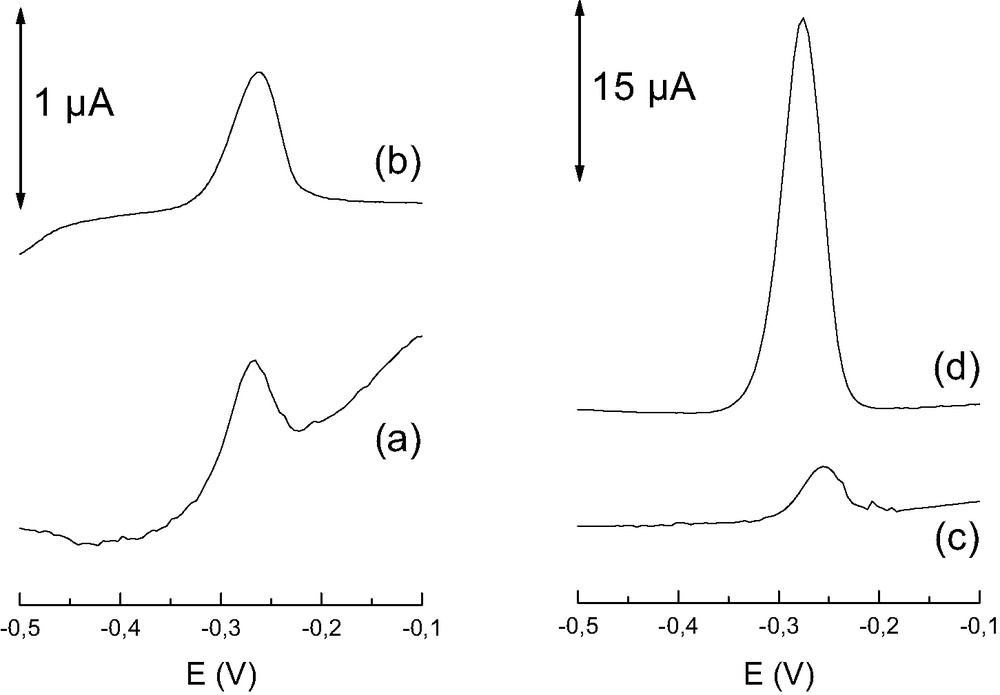
Electrochemical curves obtained after 2 min accumulation in 1 μM HgII, with various mercaptopropyl-grafted silica samples incorporated in carbon paste electrodes, (a) small pore (~4 nm) silica gel, (b) large pore (~6 nm) silica gel, (c) small pore (~3 nm) MCM-41, and (d) large pore (~6 nm) SBA-15. The curves were recorded after transfer to an analyte-free electrolyte solution (5% thiourea in 0.1 M HCl), by applying anodic stripping voltammetry in the differential pulse mode. From Ref. [82].
Electrochemical sensors made of functionalized MPS modified electrodes were also developed by the Lin group [83,84,106]. Carbon paste electrodes modified with either thiol or carbamoylphophonic acid self-assembled monolayer on MPS were used for the simultaneous determination of Hg2+ and Pb2+ [83] and Cd2+, Cu2+ and Pb2+ [84]. Detection limits in the ppb concentration range were achieved, with better selectivity than that reported for other commercially-available electrode modifiers as ion exchange resins. MPS thin films with thiol-terminated monolayer were also generated on gold electrode array and applied to the adsorptive stripping analysis of Pb2+ [106]. Interestingly, these devices exhibited long-term operational stability, low maintenance requirements, and reproducible responses. High sensitivity and large dynamic range for metal ion sensing was also achieved due to high binding site density and open mesostructure. Note that the electrode configuration was found to affect the sensitivity of the preconcentration analysis. As for zeolite-modified electrodes [133], faster response time and lower detection limits were obtained with the modified carbon paste electrodes with respect to the nanoengineered film-based sensors [83,106]. Indeed, the film could act as a physical barrier between the solution and the electrode surface, which is likely to limit somewhat diffusion of the analyte from the solution through the functionalized coating to the electrode surface.
Other functionalized MPS were used to modify carbon paste electrodes. Mesoporous cation exchangers bearing sulfonic acid moieties were prepared by oxidation of the mercaptopropyl-functionalized silica spheres obtained by the co-condensation route with various thiol group contents [86] (some pristine materials are illustrated in Fig. 9). After dispersion of them into carbon paste, the corresponding electrodes were investigated in ion exchange voltammetry (preconcentration-voltammetry experiments involving the accumulation of the target analyte by ion exchange [134]). The results have revealed a dramatic influence of the materials structure and functionalization level on the electrochemical response to Cu2+ and Ru(NH3)63+ [86]. Again, the highest sensitivity was obtained with the materials meeting the best compromise between a high density of organofunctional groups and sufficiently high porosity to maintain an easy and fast access of guest cations to the ion exchanging sites. Another example concerns the preparation of surfactant-templated MPS spheres functionalized with carnosine moieties (dipeptide β-alanyl-l-histidine) [85]. Similarly to their amorphous analogues [134], these materials can be incorporated into carbon paste electrodes and applied to the voltammetric detection of Cu2+ (known to bind selectively to the carnosine ligand), with better sensitivity for the ordered solids [85].
7 Electrocatalysis
Designing chemically modified electrodes for electrocatalysis [135–138] has been extensively developed because it provides an elegant way to facilitate (accelerate) charge transfer processes. This contributes to decrease the overpotentials often required to perform electrochemical transformations as well as to increase the intensity of the corresponding voltammetric responses. From this point of view, silica-based materials have been largely used as supports for several kinds of electrocatalysts (charge transfer mediators, metal or metal oxides layers or nanoparticles, polyoxometalates, …) [17,25–27]. Till now, electrocatalytic studies with MPS-based materials are only beginning to be explored [87,98,101].
Li et al. [87] have encapsulated 1:12 phosphomolybdic acid (PMo12) in the mesopore channels of MCM-41 materials, either unmodified or grafted with amine groups. Effective heteropolyacid immobilization was achieved with stronger binding of PMo12 to the amine-functionalized silica because of favorable electrostatic interactions induced by the acid–base equilibrium (Eq. 5), in comparison to the pure silica MCM-41.
(5)
After incorporation into carbon paste electrodes, these materials were found to be efficient catalysts for the reduction of ClO3– and BrO3– species, displaying long-term stability [87]. No comparative data were provided in that article to highlight the eventual beneficial effects due to the ordered mesoporous structure with respect to the corresponding PMo12-doped amorphous silica-based sol-gel materials, which were used otherwise for that purposes [139].
The electrocatalytic properties of 4,4′-bipyridinium units covalently attached to MCM-41 silica walls were also described with respect to the oxidation of 1,4-dihydrobenzoquinone [101]. The material denoted BP-MCM-41 was obtained via co-condensation of TEOS with a silylated precursor prepared by attachment of bipyridine moieties to trimethoxysilylpropyl groups [140]. In agreement with the behavior of cobalt complexes strongly bound to the MCM-41 mesopore walls [92], and unlikely to what happened for encapsulated species having some freedom of movement in the channels (see Section 4), the electrochemical response of BP-MCM-41 was confined to a shallow boundary zone of the particles (i.e., restricted to bipyridinium moieties located at the entry of the mesopores).
Mesoporous molecular sieves were also used as supports for metallic nanoparticles: Pd2+ species were first embedded in SBA-15, which were then electrochemically reduced to Pdn0 in the presence of CO, being suitable for electrocatalytic applications [98]. Of related interest is the ruthenium-modified MCM-41 mesoporous molecular sieve applied to the selective hydrogenation of cinnamaldehyde [141] and the electrocatalytic activity for methanol oxidation at colloidal-Pt-modified carbon-silica composite aerogels [142].
8 Bioelectrochemistry
The field of bioelectrochemistry involving ordered mesoporous solids is also rather young. It began with a work by Liu et al. [143] who have created mesoporosity in zeolite Y by hydrothermal treatment, prior to immobilization of glucose oxidase enzymes in the mesoporous host thus obtained. They have then integrated the resulting material to an amperometric oxygen detector and applied the whole device to the electrochemical biosensing of glucose. In the same time, Cosnier et al. [144,145] have described the interest of mesoporous TiO2 films to support the same enzymes, immobilized in either polypyrrole or a laponite gel-glutaraldehyde composite matrices, leading to the sensitive detection of the enzymatically-generated hydrogen peroxide at low overpotentials thanks to the electrocatalytic properties of TiO2.
Later on, cytochrome c was immobilized into the mesoporous molecular sieves MCM-48 and SBA-15, by simple impregnation, which was followed by silylation of the mesopore openings to prevent leaching of the biomolecule in the external solution [93]. Upon encapsulation, cytochrome c was found to retain its redox activity but the voltammetric signals were rather small, indicating that only those biomolecules located at the outermost surface of MCM-48 and SBA-15 particles were electrochemically accessible. Positive potential shifts were observed with respect to the redox behavior of the protein in solution, suggesting the existence of interactions with the surface of the molecular sieve host and possible conformational changes upon entrapment. These shifts were greater for the small pore (32–35 Å in diameter) MCM-48 sample of higher protein loading, in comparison to the less filled SBA-15 material characterized by larger pore size (~100 Å), which is fully compatible with the above hypothesis. Finally, the direct electron transfer and enzymatic activity was claimed for hemoglobin immobilized in a hexagonal MPS matrix and, after overcasting onto the surface of a glassy carbon electrode, the resulting electrochemical biosensor being successfully applied to the amperometric detection of hydrogen peroxide and nitrite ions in the μM concentration range [99].
9 Gas sensors
MPS thin films prepared by dip-coating of a precursor solutions containing TEOS and CTAB on Si or Al2O3 substrates with gold-array interdigitated electrodes [108–111]. After template extraction by calcination, the resulting devices were applied to the amperometric sensing of water [108,111] or alcohol [109,110] vapors, with rather good sensitivity due to the easy access of the mesoporous network from the external environment. A NO gas sensor was designed from a self-ordered cubic-like MPS film integrated in a metal-insulator-semiconductor (Au/SBA-16/Si3N4/SiO2/Si) device, based on surface photovoltage measurements, for which the good performance was attributed to the attractive characteristics of the SBA-16 material (large surface area and bi-continuous mesoporous structure) [107]. CO, H2 and CH4 gas sensors were also developed from pressed pellets of SnO2-MCM-41 mixtures comprising two Pt wires directed to measuring resistance changes arising from the presence of the target gaseous analytes [95].
10 Conclusions
MPS-based materials offer attractive features that can be readily exploited in electrochemistry (mesostructural order, very high specific surface area, sorption and ion exchange properties, and numerous possibilities of functionalization by covalent anchoring of organic or organometallic groups, among others). At this stage, however, the intersection between the chemistry of MPS and electrochemical science is rather young, and researchers are just excited to point out the feasibility of selected applications involving mesoporous materials in an electrode device, or to demonstrate that some electrochemical techniques can help at characterizing the materials properties and/or the redox activity of encapsulated species. Anyway some interesting achievements have been already reported and the field is expected to grow quite rapidly in exciting (new or emerging) directions on the basis of the huge developments of zeolite- and (organo)silica-modified electrodes, since MPS exhibit molecular sieving properties and long-range order, similarly to zeolites, associated to the surface reactivity of silica.
Summarizing the field of electrochemical applications using MPS-based materials, it appears that these molecular sieve adsorbents (organically functionalized or not) act as active hosts when immobilized on solid electrode surfaces and have advantage of ensuring fast mass transfer rates due to their regular mesostructure. They were successfully exploited in voltammetric analysis of heavy metal ions subsequent to open-circuit preconcentration, for electrocatalytic purposes, as hosts for biomolecules in the biosensors field, and in gas sensing devices. In turn, voltammetric methods have been used to get information on the activity of redox probes incorporated within these mesoporous matrices and to characterize the speed at which guest molecules or ions are reaching the active centers in mesoporous organic–inorganic hybrids for which significant enhancement was observed in comparison to the correspondingly functionalized amorphous silica gels.