1 Introduction
Partial oxidation of methane (POM) (reaction (1)), to produce syngas, is a process that is still very much studied for many advantages compared to steam reforming of CH4. It is an exothermic reaction and produces syngas with H2/CO ratio of about 2 which is ideal for further methanol and Fischer–Tropsch syntheses [1–4].
CH4 + 1/2O2 → CO + 2H2; ΔH298 K = −35.6 kJ mol−1 | (1) |
Although precious metals, such as Ru, Rh, Ir and Pt, have been employed successfully for this reaction in terms of activity and selectivity [5,6], the high cost and limited availability of the noble metals restrict their applications. On the other hand, nickel catalysts have been widely used due to their high activity and low cost. However, most commercial nickel-based catalysts suffer deactivation due to sintering and carbon deposition during operation [7,8]. Catalysts that are more resistant to deactivation have been developed by nickel insertion into a well-defined structure, such as perovskites, spinels,…, the strong interaction existing within the structure leads to the formation of small nickel particles upon the reduction of the catalyst: which resulted in a high dispersion of the active species [9,10]. On the other hand, nickel catalysts with various additives have been reported, and other transition-metal oxides have proved to be active in the catalytic oxidation of hydrocarbons. The best catalytic performance was achieved with 3d-transition-metal oxides composed of cations in their higher oxidation state. Furthermore, it has been shown that the Cu, Co and Ni mixed oxides with a spinel-type structure are more active than the single oxides [11,12].
Mixed nickel–manganese oxides adapt two types of crystal structure: spinel (NiMn2O4) and ilmenite (NiMnO3). In the spinel structure, Ni2+, Mn2+, Mn3+ and Mn4+ ions can coexist and are distributed among the tetrahedral and octahedral spinel sites, whereas in the ilmenite structure both Ni2+ and Mn4+ are in octahedral position.
In this study, we report the preparation of NiMn2O4 using coprecipitation and sol–gel methods. Preliminary reactivity results of methane partial oxidation will be given, so that the activity and the structure of catalysts could be correlated.
2 Experimental
2.1 Catalyst preparation
2.1.1 Coprecipitation method
Nickel–manganese mixed oxides were prepared from carbonate precursors [13]. The mixed appropriate amounts of manganese and nickel nitrate solutions with Ni/Mn = 1/2 were added into a well-stirred container containing sodium bicarbonate solution at room temperature. The obtained solid was dried at 60 °C and calcined at 750 and 900 °C for 12 h in air, the heating rate was 5 °C min−1. The solids prepared by the coprecipitation method and calcined at 750 and 900 °C will be denoted, respectively, as CP750 and CP900.
Mn3O4 and the sub-stoichiometric NixMn3−xO4 (x = 0.2, 0.6, 0.8) were prepared following the same protocol and then calcined in air for 12 h at 900 °C. These solids will be denoted as CP-x-900, where x stands for the stoichiometric nickel content.
2.1.2 Sol–gel method
The nickel–manganese mixed oxide was also prepared by a pseudo-sol–gel method based on the thermal decomposition of propionate precursors [14]. The starting materials were nickel(II) acetate hydrate and manganese(II) acetate hydrate. The salts were dissolved separately in boiling propionic acid with a concentration of 0.12 mol l−1 in cation. The two boiling solutions were then mixed so as to have Ni:Mn = 1:2 and the solvent was evaporated under continuous stirring until a resin was obtained. The resin was directly calcined in air at 750 and 900 °C for 12 h. The solids prepared by the sol–gel method will be denoted as SG750 and SG900, where 750 and 900 stand for the calcination temperatures.
2.2 Technique characterization
The atomic ratio of metals was checked by atomic absorption spectroscopy. X-ray diffraction experiments were performed on a D500 Siemens diffractometer using the Cu Kα radiation (λ = 0.15406 nm). The data were collected at 0.03° with a counting time of 2 s per step, in the (2θ) range from 20° to 80°. The crystalline phases were identified by reference to the powder diffraction data (JCPDS) with the use of standard spectra software. Fourier-transform infrared (FT-IR) spectra were recorded using a Shimadzu 8400 spectrometer in KBr pellets. The spectra were the results of 64 scans obtained in the range of 4000–400 cm−1 with a resolution of 1 cm−1.
The laser Raman spectra (LRS) were recorded on a LabRam infinity laser Raman spectrometer (JY-DILOR) equipped with an optical microscope. The laser intensity (Ar+, 514.5 nm) was reduced by various filters (<1 mW) and the data were treated by Lasbpec software. The spectral resolution and the accuracy in the Raman shifts are estimated to be 2 cm−1. A tenth particle was examined for each sample to check its homogeneity.
Temperature programmed reduction experiments were performed on a 50 mg sample placed in a U-shaped quartz reactor (6.6 mm ID) using a heating rate of 15 °C min−1 from 25 to 900 °C. The reductive gas was a mixture of 3% H2 in He (total flow rate 50 ml min−1). A thermal conductivity detector analyzed the effluent gas after water trapping and permitted to quantify the hydrogen consumption.
2.3 Activity test
Activity tests were performed in a fixed bed reactor using 0.2 g of catalyst at atmospheric pressure and at the reaction temperature of 700 °C. The total flow rate is of 3.4 l h−1 with CH4/O2 = 2. No pre-treatment was used. The products and reactants were analyzed by a Perkin Elmer 5500 gas chromatograph equipped with a TCD and a carboxen packed column. In all experiments, the performances of the catalysts were evaluated with the methane conversion and the product's selectivity was calculated as follows:
- CH4 conversion (%) = (moles of CH4 converted/moles of CH4 in feed) × 100
- Selectivity of H2 (%) = (moles of H2 produced/2 × moles of CH4 converted) × 100
- Selectivity of CO (%) = (moles of CO produced/moles of CH4 converted) × 100
- Selectivity of CO2 (%) = (moles of CO2 produced/moles of CH4 converted) × 100
3 Results and discussion
3.1 Chemical analysis
The results are tabulated in Table 1. The comparison of the predicted and experimental Ni/Mn molar ratio values shows that they are very close.
Predicted and experimental Ni/Mn values as determined by atomic absorption spectroscopy.
Sample | (Ni/Mn)predicted | (Ni/Mn)experimental |
CP-0.2-900 | 0.07 | 0.08 |
CP-0.6-900 | 0.25 | 0.27 |
CP-0.8-900 | 0.36 | 0.41 |
CP900 | 0.50 | 0.53 |
SG900 | 0.50 | 0.53 |
3.2 X-ray diffraction
XRD patterns of all solids are shown in Figs. 1 and 2.

XRD spectra of calcined NiMn2O4 prepared by coprecipitation method (CP) and sol–gel method (SG); (s) spinel, (i) ilmenite, and (b) Mn2O3 bixbyite.
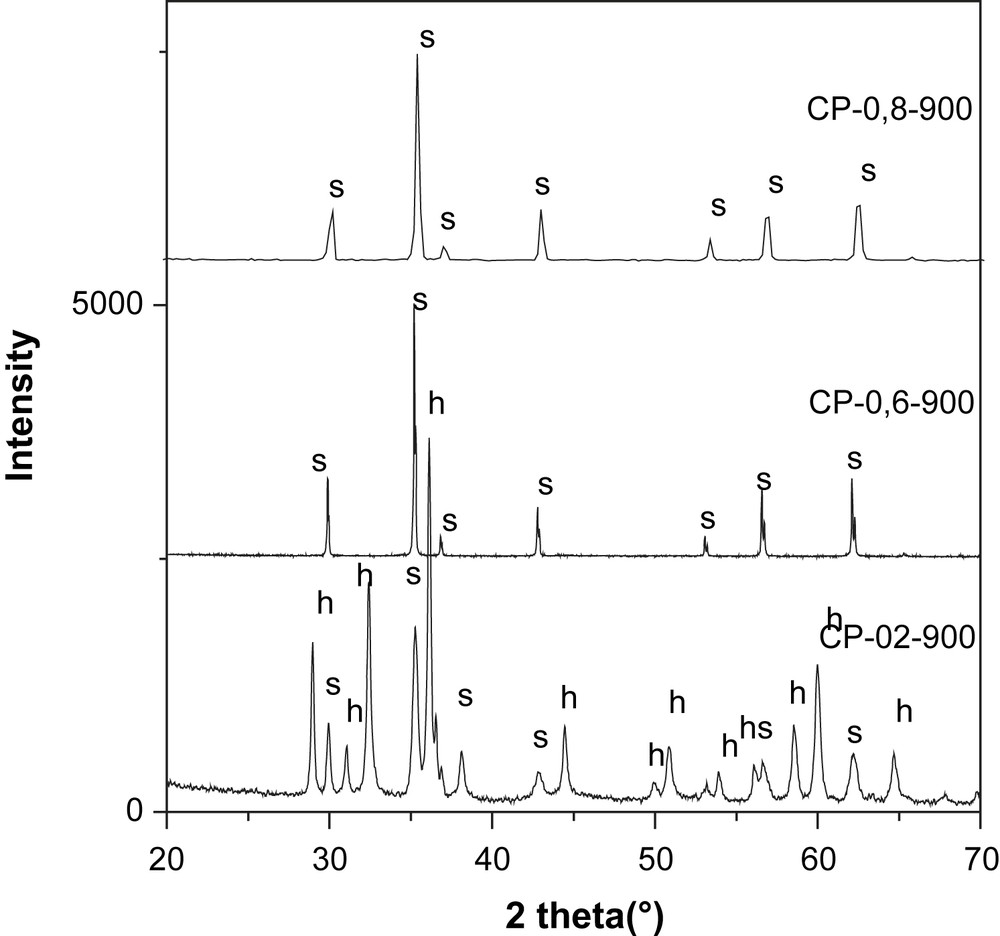
XRD spectra of the series NixMn3−xO4 (s) spinel (h) Mn3O4 hausmanite.
The patterns of the solids calcined at 900 °C show the presence of pure spinel phases for CP900, CP-0.6-900 and CP-0.8-900. Traces of Mn2O3 were observed for the solid SG900. The diffractogram of CP-0.2-900 shows the presence of the spinel phase in addition to hausmanite phase: Mn3O4.
The solids calcined at 750 °C show the presence of a mixture of three phases NiMnO3 (ilmenite), NiMn2O4 (spinel) and Mn2O3.
3.3 FT-IR spectral analysis
The IR spectra are represented in Figs. 3 and 4. The FT-IR spectra of solids prepared with sol–gel method show a broad band with a maximum centered at around 3370 cm−1, together with a band at 1630 cm−1. The band at 3370 cm−1 can be ascribed to the hydroxyl groups bonded through hydrogen bonds, whereas the band at 1630 cm−1 corresponds to the adsorbed molecular water. These results indicate that these catalysts are hydrated and/or hydroxylated.
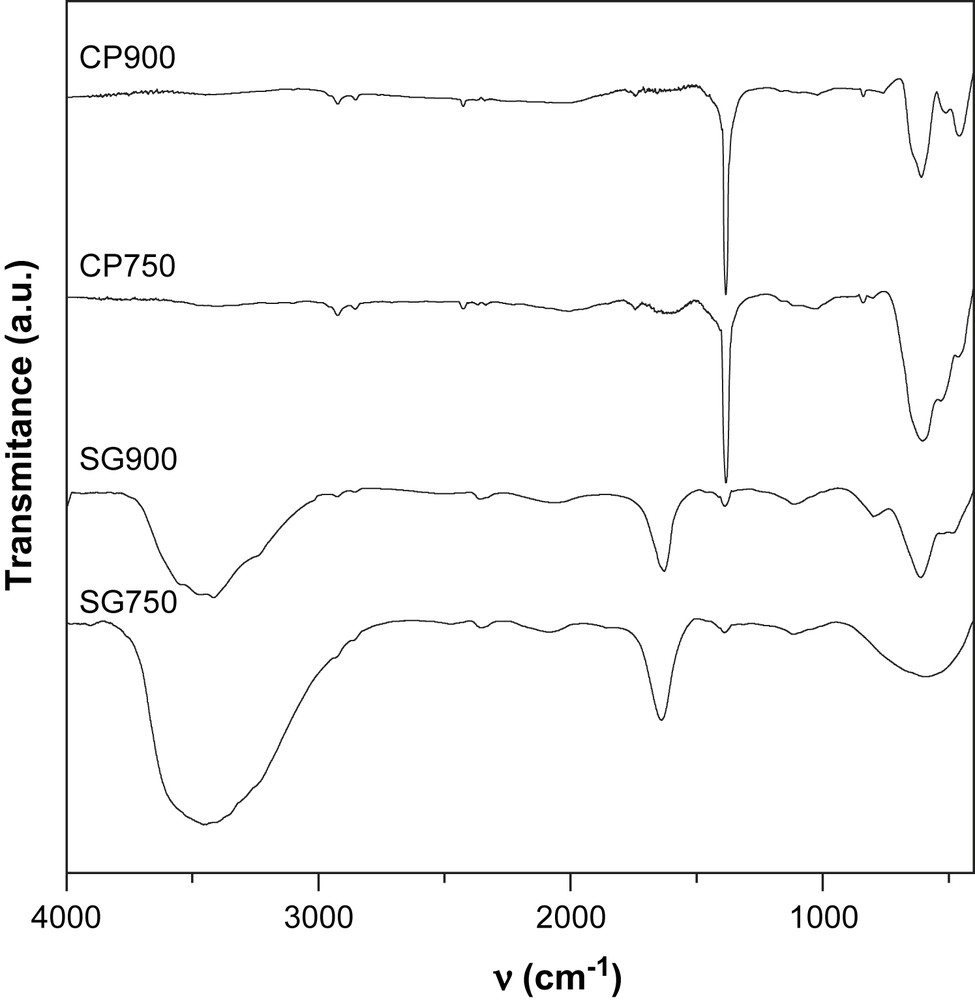
FT-IR spectra of calcined NiMn2O4 prepared by coprecipitation and sol–gel methods.
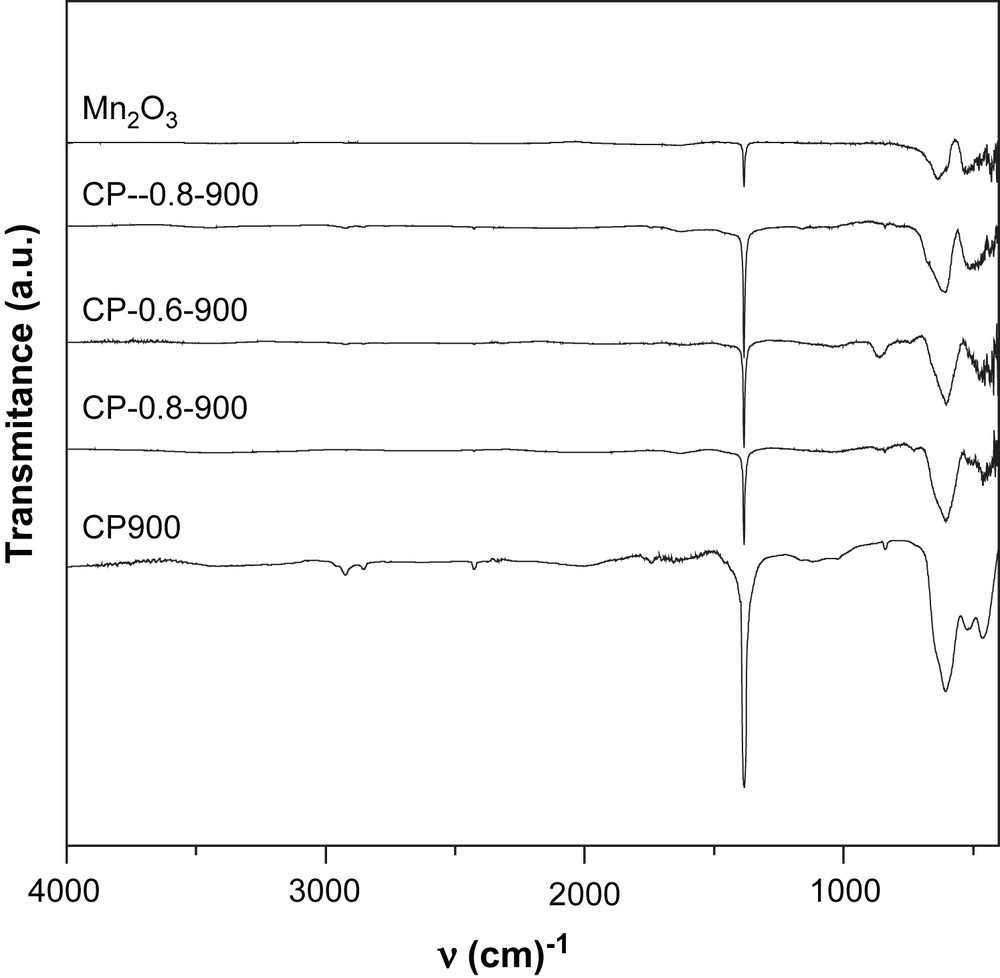
FT-IR spectra of calcined NixMn2−xO4 (x = 0, 0.2, 0.6, 0.8, 1).
All the solids prepared via the coprecipitation method exhibit a relatively intense narrow band at 1384 cm−1 which can be attributed to the nitrate vibrations. The remaining of nitrates after calcination at high temperatures is not expected. However, the presence of small quantities of sodium ions provided by the precipitation reactant (Na2CO3) may stabilize a small quantity of nitrate through the formation of sodium nitrate. This salt has been already reported to be stable in air at 850 °C [15].
In the range of 400–1000 cm−1, the IR bands of inorganic solids are usually assigned to the vibration of metallic ions in the crystal lattice. Two main broad metal–oxygen bands are seen in the IR spectra of normal spinels. The highest one, ν1, generally observed in the range of 600–550 cm−1, corresponds to the intrinsic stretching vibration of the metal ion at the tetrahedral site, Mtetra–O, whereas the ν2 lowest band, usually observed in the range of 450–385 cm−1, is assigned to the octahedral metal ion stretching, Mocta–O [16,17]. In agreement with these statements, Mn3O4 presents two bands at 436 cm−1 and 636 cm−1. However, three bands in the range of 450–600 cm−1 are observed in the spectra of the solids calcined at 900 °C which seem to indicate a partial or total inversion of the spinel NiMn2O4. Indeed, in the inverted spinel, octahedral sites are occupied by two types of ions which might result in the doubling of the band corresponding to the vibration of Mocta–O.
For the solids calcined at 750 °C, the appearance of more than two bands in the range of 400–1000 cm−1 is attributed to the presence of mixed oxides formed at this temperature as shown by XRD.
3.4 Temperature programmed reduction
The TPR profiles for the solids CP750, CP900, SG750 and SG900 are presented in Fig. 5. For all oxides the reduction occurs practically in two main steps. The TPR curves of the coprecipitated catalysts (CP750 and CP900) show two main peaks situated at around 443–460 °C and 512–517 °C, the reduction peaks of the solid calcined at 900 °C are slightly shifted to higher temperatures. Additional unresolved peaks, at higher reduction temperature, are observed in the range of 560–850 °C.
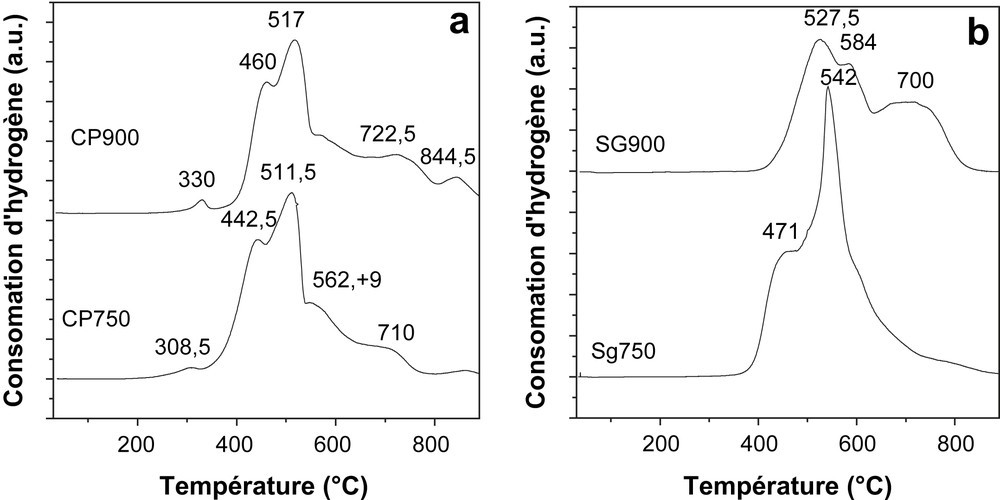
Temperature programmed reduction profiles of calcined NiMn2O4 prepared by (a) coprecipitation method and (b) sol–gel method.
The sol–gel solids exhibit different TPR profiles compared to the solids prepared by coprecipitation. Moreover, the two solids show two different profiles, indeed, the solid calcined at 750 °C shows two reduction steps where the second one consists of one single sharp peak, meanwhile, for the solid calcined at 900 °C, the main reduction step is the first one in opposite to the three other solids.
The total hydrogen consumption was practically the same for the four samples and seemed not to depend on either the calcination temperature or the preparation method.
XRD analysis of the solids after TPR experiments showed that all of them consisted of a mixture of MnO and metallic nickel. These results are in agreement with the data reported in the literature [18] where it has been shown that the reduction of nickel manganites (spinel and ilmenite) occurs in two stages:
NiMn2O4, NiMnO3 → NiO + MnO → Ni + MnO |
During the first stage, the major part of manganese cations are reduced to Mn2+, leading to the formation of a solid solution NiO–MnO, the reduction of Ni2+ cations in MnO matrix occurs during the last step. According to these attributions, the reduction of nickel in our solids seems to be difficult, in that way that it occurs very slowly, giving rise to broad shoulders on the second reduction step peak; the unusual TPR profile obtained for the solid SG750 seems to indicate that the reduction of all the present nickel ends at 700 °C.
A small peak is observed at 309 and 330 °C on the profiles of CP750 and CP900, respectively, it can be attributed to the reduction of small free nickel oxide which could have segregated from NiMn2O4 and NiMnO3. The reduction of NiO has already been reported to occur in the range of 307–370 °C [19].
3.5 Raman spectroscopy analysis
Raman spectra are given in Fig. 6. CP900 and SG900 spectra exhibited two distinct bands, a weak band at about 516 cm−1 and a stronger one at about 645 cm−1.
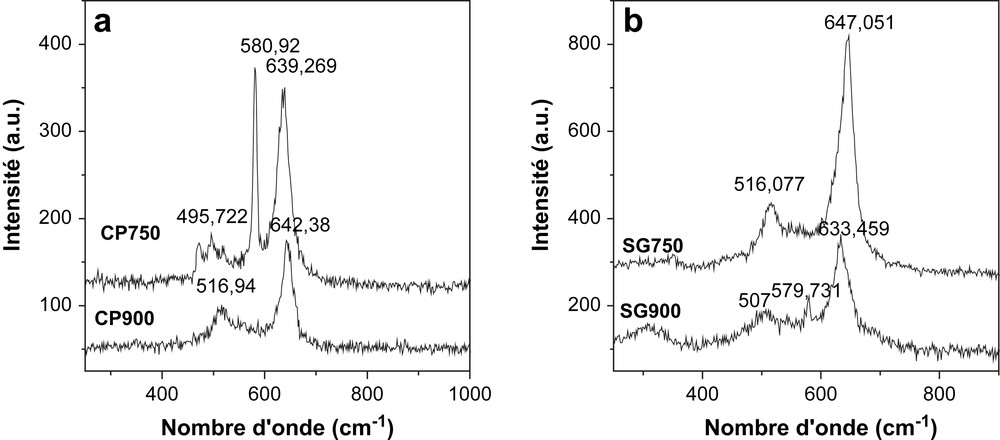
Raman spectra of calcined NiMn2O4 prepared by (a) coprecipitation method and (b) sol–gel method.
In spinel oxides and in other manganese oxides energies of 600–650 cm−1 are characteristic of vibrations involving motion of oxygens inside the octahedral unit MnO6 [20]. The broadening of this peak in the direction of lower energies corresponds to a spinel with disordered cationic occupation (inverted spinel) [21]. The second peak, centered at around 516 cm−1 has already been observed and has been considered as an F2g mode related to M–O in tetrahedral environment [21].
For SG750 and CP750, the same two bands are preserved and a supplementary band situated at about 580 cm−1 appeared. This Raman feature is assigned as an A1g mode for regular MnIVO6 octahedra [20]. This band might, then, correspond to the manganese in the ilmenite structure as this band was absent from the Raman spectra of the solids calcined at 900 °C, this band is more intense in the solid prepared via the coprecipitation method which indicates that the ilmenite is more important in this solid than in the solid prepared via sol–gel method.
3.6 Catalyst testing
In the catalytic experiments, the catalyst is heated from ambient temperature to 700 °C under reaction mixture. The first analysis of the effluent gases is performed when the reaction temperature reaches 700 °C, the methane conversion is then very low and increases as the reaction time increases as shown in Fig. 7. The methane conversion, the products' selectivities measured at the steady state and the thermodynamic selectivities are shown in Fig. 8.
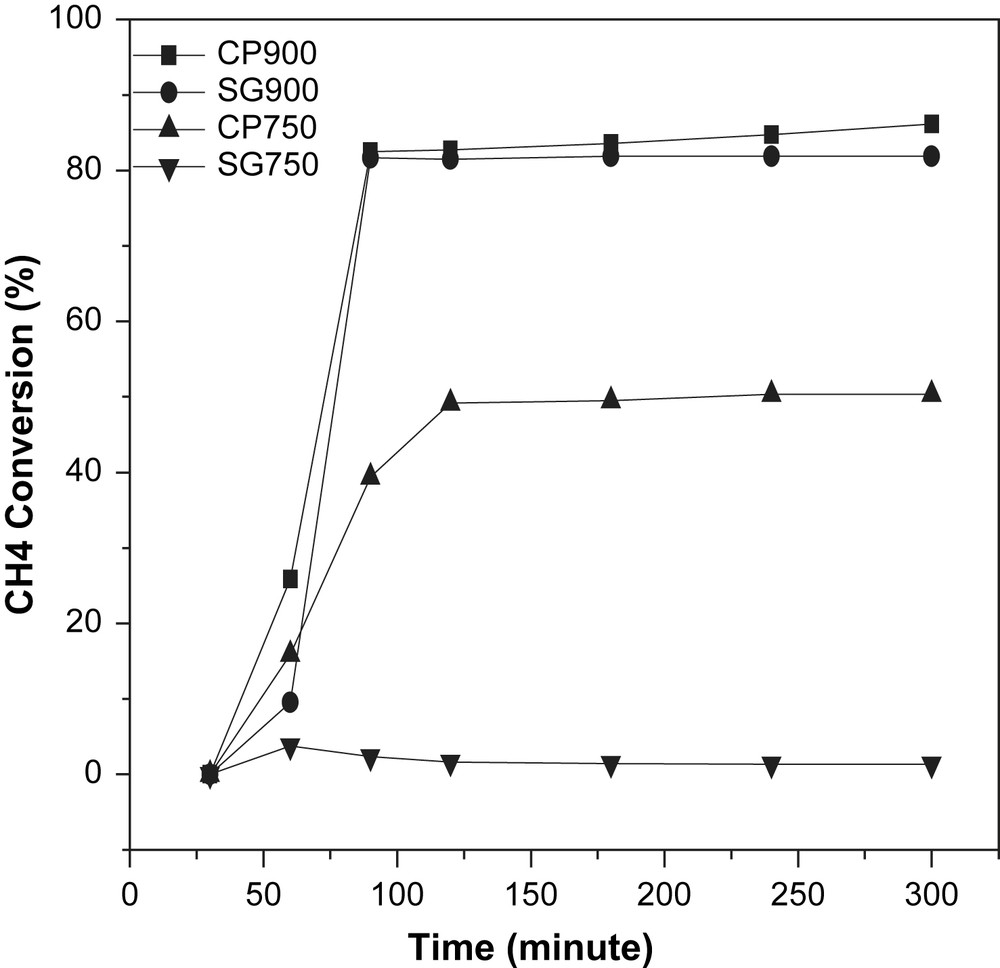
Influence of time on stream on the methane conversion at 700 °C, S.V.: 52 900 l kg−1 h−1.

Methane conversion, CO, H2 and CO2 selectivities at the steady state. Reaction temperature: 700 °C, S.V.: 52 900 l kg−1 h−1.
The catalysts CP900 and SG900 displayed high and similar activities towards the partial oxidation of methane characterized by a CH4 conversion of about ∼80%.
Meanwhile, the solids CP750 and SG750 showed different behaviors, indeed, the solid prepared with coprecipitation method showed a methane conversion of 51% whereas the sample prepared via the sol–gel method presented a very poor activity (about 1% of CH4 converted).
Further examination of Fig. 8 let us to remark that the three active catalysts showed good selectivities towards CO and H2 and that the solid CP750 leads to a high CO2 selectivity. This fact may be related to the presence of the ilmenite phase (NiMnO3) which has been already proved to have excellent catalytic activity in complete hydrocarbon oxidation attributed to the manganese present in its higher oxidation state.
In order to understand the lack of catalytic activity in the case of SG750, we compared the XRD patterns obtained with the used solids (shown in Fig. 9). We can see that the used SG750 consisted, practically, of a mixture of MnO and metallic nickel which indicated that the solid undergoes deep reduction under the reaction conditions. This is consistent with the exceptional reduction behavior of this solid already observed in TPR measurements. In the same time, the XRD patterns of the used active catalyst (e.g. CP750) showed that only a part of nickel is present as metallic phase with a size particle of 27.12 nm whereas the size particle of metallic nickel in the case of SG750 is about 5.29 μm. In the light of these observations, we propose that the inactivity of the solid SG750 is due to the sintering of the active phase and the high activity of CP750 is the consequence of the good dispersion of nickel particles.
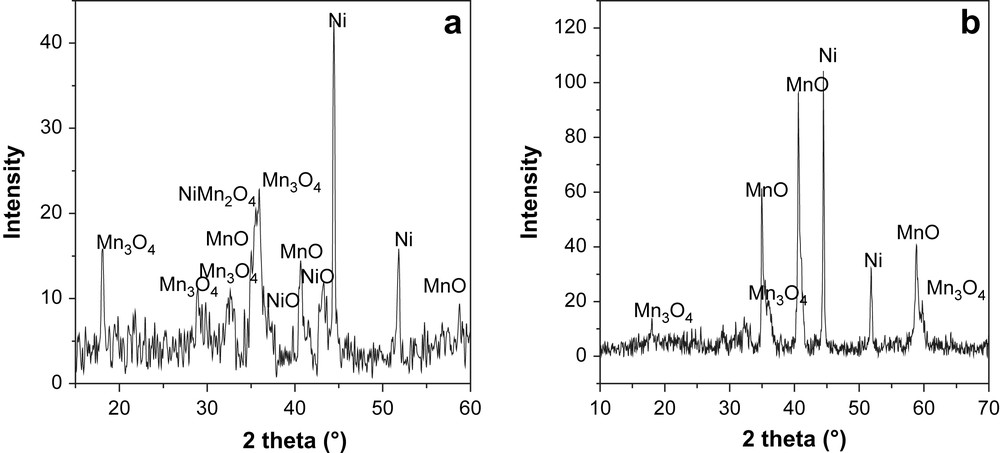
XRD patterns of catalysts after methane partial oxidation (a) CP750, (b) SG750.
The effect of nickel content on the catalytic activity is summarized in Table 2. We have found out that the catalysts corresponding to compositions NixMn2−xOy (x < 1) exhibited very low methane conversion at 700 °C. In Table 2, we are giving the temperature at which a methane conversion of about 80% had been achieved. It appears that the decrease in nickel content caused the increase in the iso-conversion reaction temperature. This observation is in agreement with the fact that nickel is considered as the active species in POM.
Effect of the composition of the catalysts on the conversion of CH4.
X | Conversion (%) | Iso-conversion temperature (°C) |
0.2 | 78 | 850 |
0.6 | 82.8 | 800 |
0.8 | 79 | 750 |
1 | 82.7 | 700 |
4 Conclusion
NiMn2O4 was prepared by sol–gel and coprecipitation methods, a pure phase was obtained after calcination at 900 °C. FT-IR and Raman techniques illustrated that the synthesized spinels were inverted. The calcination at 750 °C led to the formation of NiMnO3 and NiMn2O4 mixture. A pure spinel was also obtained at 900 °C with the sub-stoichiometric solids Ni0.6Mn2.4O4 and Ni0.8Mn2.2O4.
The spinel NiMn2O4 was found to be active in the reaction of the partial oxidation of methane. The catalysts calcined at 900 °C present a higher methane conversion; the reason can be the stability of the structure which led to good dispersion of nickel species. Indeed, the presence of the oxidized nickel limited the growth of the particles probably by the formation of interaction between metallic nickel out of the structure and the nickel oxide of the structure.
The catalytic activity of the ilmenite nickel–manganese in the complete oxidation of methane can be explained having in mind that, in the ilmenite structure, manganese ions are stabilized in a high oxidation state (Mn4+). Evidently, for nickel–manganese oxide catalysts, Mn4+ ions were responsible for the complete oxidation reaction.