1 Introduction
Nanoscale materials are being pursued extensively because of their crystal structures, optical, magnetic, electrical and catalytic properties, which are strongly composition-, structure-, size- and shape-dependent [1–12]. It is known that metal sulfides exhibited interesting electronic properties and achieved several technological applications [13]. Among the family of metal sulfides, nickel sulfides have attracted much attention owing to their potential applications as a transformation-toughening agent for materials used in semiconductor applications [14], catalysts [15], and cathode materials for rechargeable lithium batteries [16]. Depending on the synthetic process, a variety of compositions can be obtained, including Ni3S2, Ni3+xS2, Ni4S3+x, Ni6S5, Ni7S6, Ni9S8, Ni3S4, and NiS. NiS exhibits two phases: the low-temperature rhombohedral (b-NiS, millerite) and high-temperature hexagonal (α-NiS) crystal structures [17]. Nickel sulfide was prepared by different methods, such as high-temperature solid-state reaction and vapor phase reaction [18,19], hydrothermal [20], and solvothermal [21]. A suitable method for the synthesis of nanoparticles is microwave radiation [22,23].
Our strategy was the use of thioacetamide (TAA) as a capping agent and sulfur source for microwave radiation in synthesis of NiS nanoparticles. It still remains a great challenge to develop facile and environmentally benign methods for creating NiS nanoparticles from a simple precursor. A major interest at the moment is in the development of organometallic or inorganic compounds for the preparation of nanoparticles. Using of novel compounds can be useful and open a new way for preparing nanomaterials to control nanocrystal size, shape and distribution size. Previously, our research team has reported synthesis of different metal sulfide nanostructures by using thioglycolic acid as capping agent [24–26]. To continue, in this paper we investigate different conditions in order to achieve NiS nanoparticles. To the best of our knowledge, there are no reports on the preparation of NiS nanostructures by nickel complex via the microwave radiation method.
2 Experimental
2.1 Materials and physical measurements
All chemical reagents in this experiment were of analytical grade and used without further purification. X-ray diffraction (XRD) patterns were recorded by a Philips-X’pertpro, X-ray diffractometer using Ni-filtered Cu Kα radiation. Fourier transform infrared (FT-IR) spectra were recorded on the Nicolet Magna-550 spectrometer in KBr pellets. The electronic spectra of the sample were taken on a Perkin-Elmer LS-55 luminescence spectrometer and a UV–Vis scanning spectrometer (Model 2101 PC). Scanning electron microscopy (SEM) images were obtained on LEO-1455VP equipped with an energy-dispersive X-ray spectroscopy (EDX). Field Emission Scanning electron microscopy (FESEM) images were obtained on HITACHI S-4160.
2.2 Preparation of Ni(HAP)2 as a precursor
At first, 6 g of Ni(OAC)2 were dissolved in 50 ml of ethanol. Then, 8.2 ml of 2-hydroxy acetophenone were dissolved in 20 ml of ethanol. Finally a solution of 2-hydroxy acetophenone was slowly added to a solution of Ni(OAC)2 and the final solution was stirred for 24 h [27]. In this investigation, Ni(HAP)2 was used as a Ni ions source.
2.3 Prepration of NiS nanoparticles
First, 0.2 g of Ni(HAP)2 was dissolved in 30 ml of ethylene glycol (EG) and 0.137 g TAA was dissolved in 10 ml of EG separately and added into the solution of Ni(HAP)2. The solution was stirred at ambient temperature (for 1 h). Then, the reaction was carried out in a microwave irradiation for five cycles. Each cycle was 30 s on and 60 s off. Then, the products were washed with water and methanol, dried at 50 °C for 12 h. Reaction conditions are presented in Table 1 and schematically shown on Scheme 1.
Experimental condition for preparation of NiS.
Sample No. | Ni ion (g) | S (g) | Ni:S | Solution | Time (min) | Power (W) | Source of sulfide |
1 | 0.2 | 0.137 | 1:3 | EG | 2.5 | 600 | TAA |
2 | 0.2 | 0.045 | 1:1 | EG | 2.5 | 600 | TAA |
3 | 0.2 | 0.09 | 1:2 | EG | 2.5 | 600 | TAA |
4 | 0.2 | 0.18 | 1:4 | EG | 2.5 | 600 | TAA |
5 | 0.2 | 0.137 | 1:3 | EG | 5 | 600 | TAA |
6 | 0.2 | 0.137 | 1:3 | EG | 10 | 600 | TAA |
7 | 0.2 | 0.137 | 1:3 | EG | 15 | 600 | TAA |
8 | 0.2 | 0.137 | 1:3 | EG | 2.5 | 300 | TAA |
9 | 0.2 | 0.137 | 1:3 | EG | 2.5 | 900 | TAA |
10 | 0.2 | 0.137 | 1:3 | PEG | 2.5 | 600 | TAA |
11 | 0.2 | 0.137 | 1:3 | Water | 2.5 | 600 | TAA |
12 | 0.2 | 0.138 | 1:3 | EG | 2.5 | 600 | TU |
13 | 0.2 | 0.288 | 1:3 | EG | 2.5 | 600 | Na2S2O3 |
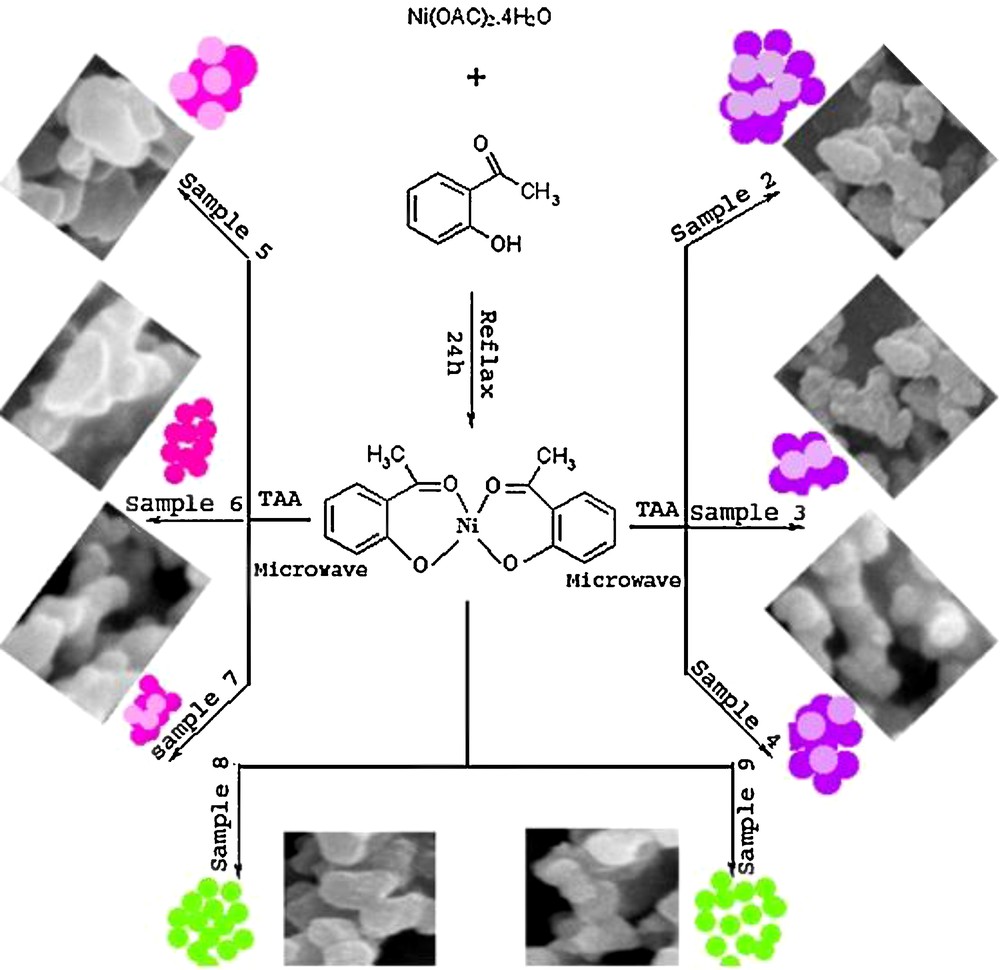
Reaction condition for the preparation of as-synthesized products.
3 Result and discussion
The crystal structures of the as-prepared products have been identified. As shown on Fig. 1, the intense and sharp diffraction peaks suggest that the obtained product is well crystallized. All the diffraction peaks can be indexed as the pure NiS phase (JCPDS Card No. 12-0041). Also, it can be concluded that the obtained samples were small and in nanosize due to the broadening of the diffraction peaks. No remarkable diffractions of impurities such as Ni, NiOx in the XRD pattern were observed, indicating that a pure NiS phase has been formed.

X-ray diffraction pattern of NiS nanoparticles.
Ni(HAP)2 and TAA were mixed in EG and stirred at ambient temperature. The formation mechanism for this synthesis is schematically presented in Scheme 2. It can be seen that as the reaction processed, the bonds between HAP and Ni are dissociated and then TAA forms a complex with Ni via its NH2 group. Then, this complex is ruptured under microwave irradiation and Ni ions are released in the reaction medium. The as-released TAA hydrolysis and produce H2S, which reacts with Ni ions and NiS is produced.
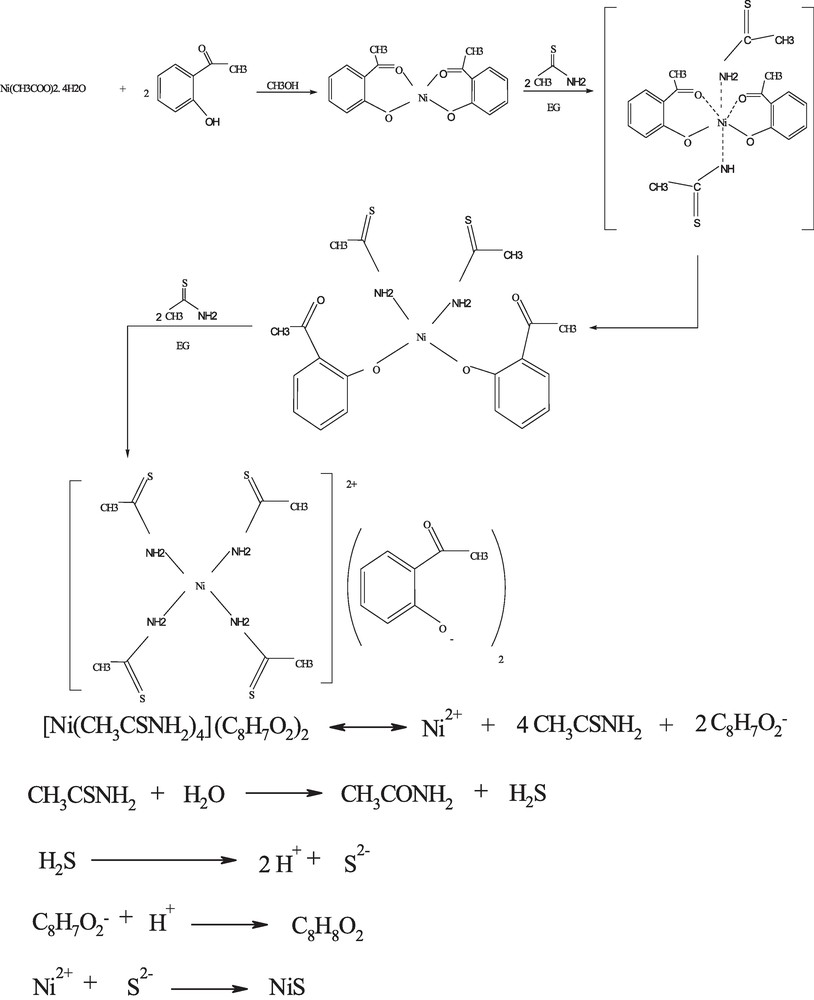
Synthesis of NiS nanoparticles.
The FT-IR spectrum of the as-synthesized NiS is shown in Fig. 2. It shows impurities that can arise from TAA absorbed on the surface of the sample. The peak at 3429 cm−1 can be ascribed to the absorption of H2O in the sample. Two weak peaks at 2920 and 2885 cm−1, respectively, are due to C–H stretching modes of the TAA carbon chain, indicating that TAA molecules are absorbed on the surface of nickel sulfide nanoparticles. There was no evidence of the free precursor, [Ni(HAP)2], in the sample, because the stretch vibration of C–H (yC–H) and that of CO and CC benzene ring disappeared.
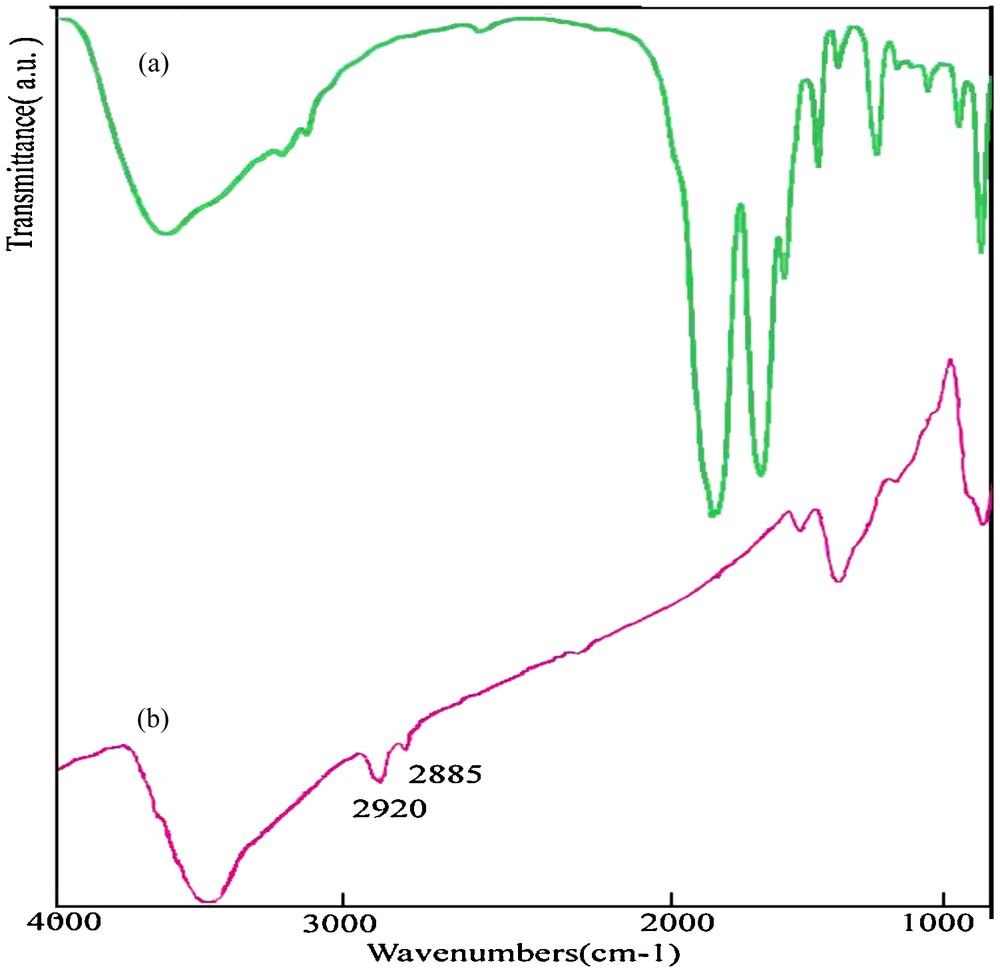
FT-IR of the as-synthesized products: (a) [Ni(HAP)2] and (b) NiS nanoparticles.
The optical properties of NiS nanoparticles obtained in pure ethanol were investigated at ambient temperature using UV–vis absorption spectroscopy (Fig. 3a). For NiS nanocrystals, the UV–vis absorption spectra display two absorption peaks located at 273 nm and 224 nm, respectively. The absorption spectra show a blue shift compared to that of the bulk NiS (approximately 2.1 eV) [28]. These amounts of blue shift for a-NiS and Ni3S4 nanoparticles may be attributed to the low dimension of the particles [29]. Fig. 3b shows the PL spectrum of the as-synthesized NiS nanoparticles (excitation at 340 nm) at room temperature. The PL spectrum of the NiS nanoparticles shows a peak centered at 555.04 nm. Different morphologies of nanoparticles of nickel sulfide may be responsible for this PL spectrum, though the detailed reasons are unclear at present.

(a) UV–vis absorption and (b) PL spectra of the as-prepared NiS nanoparticles.
Fig. 4 shows the magnetization versus applied magnetic field (M–H) curve for the as-synthesized NiS nanoparticles. As expected, the M–H curve reveals a weak paramagnetic behavior with remnant magnetization (Mr) and coercivity (Hc) values of ca. 0.0046 emu/g, and 273.21 Oe, respectively.

Magnetization curve of NiS nanoparticles.
The local elemental composition of the as-formed nanoparticles was studied by EDX microanalysis as shown in Fig. 5. It confirms that the nanoparticles are composed of Ni and S. A little amount of oxygen was determined in this analysis which may be due to the oxidation of a low amount of the product in the air.
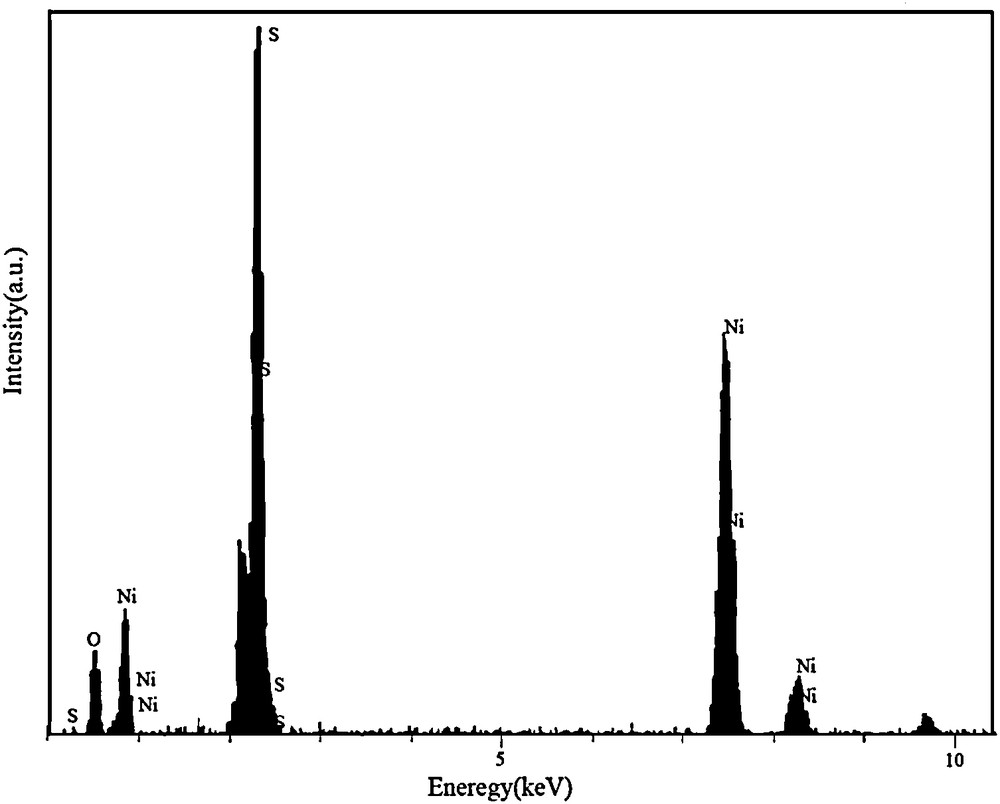
Energy dispersive X-ray spectrum of NiS nanoparticles.
On Fig. 6, SEM images of NiS nanoparticles (sample No. 1) are shown. These images reveal that the morphology of the products is quasi-spherical.
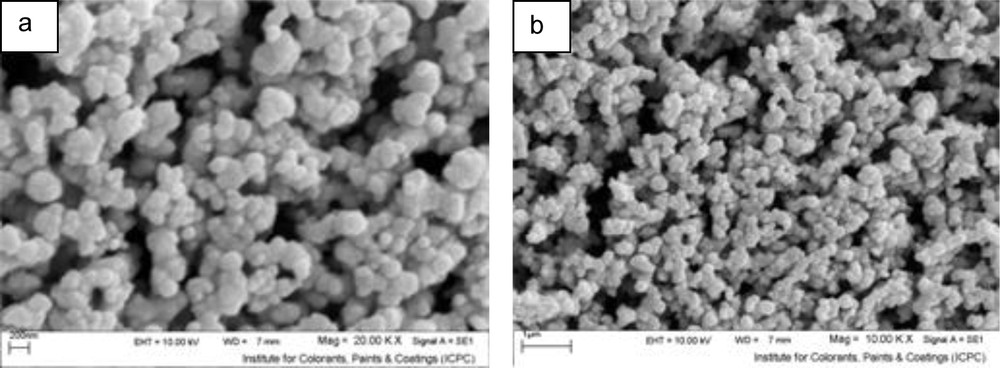
Scanning electron microscopy images of the products prepared using Ni(HAP)2 and thioacetamide at 1:3 molar ratio, 2.5 min inserted by microwaves at 600 W (sample No. 1).
Fig. 7 shows the relation between the size of the particles and the concentration of nickel ions and TAA. It was observed that when concentration of TAA increased, the size of the particles decreased and the optimum conditions were obtained at the molar ratio was 1:3 (Ni2+:TAA). It is believed that when the reactants ratio increased, the nucleation rate increased, which led to the formation of small nuclei at the same time. Further increasing of this ratio may lead to agglomeration due to the formation of unstable and ultra-fine nuclei that have a strong tendency to be stable by agglomeration. Fig. 8 indicates the relation between particle size and time of reaction. Three different reactions were carried out; in the first reaction, the process was done in 10 cycles (30 s on and 60 s off) while in the second and third reaction, reactions were carried out in 20 and 30 cycles (30 s on and 60 s off), respectively. It was observed that when the time was increased to 5 min, the size of the particles increased and they were agglomerated, but in 10 or 15 min, the size of nanoparticles decreased, which may be due to a better separation of agglomerated nuclei at higher reaction time. Nevertheless, when the time of reaction was 2.5 min, the size of particles was smaller. Indeed, the optimum time for the reaction was 2.5 min and it can be concluded that with increasing the reaction time, the particles were agglomerated.
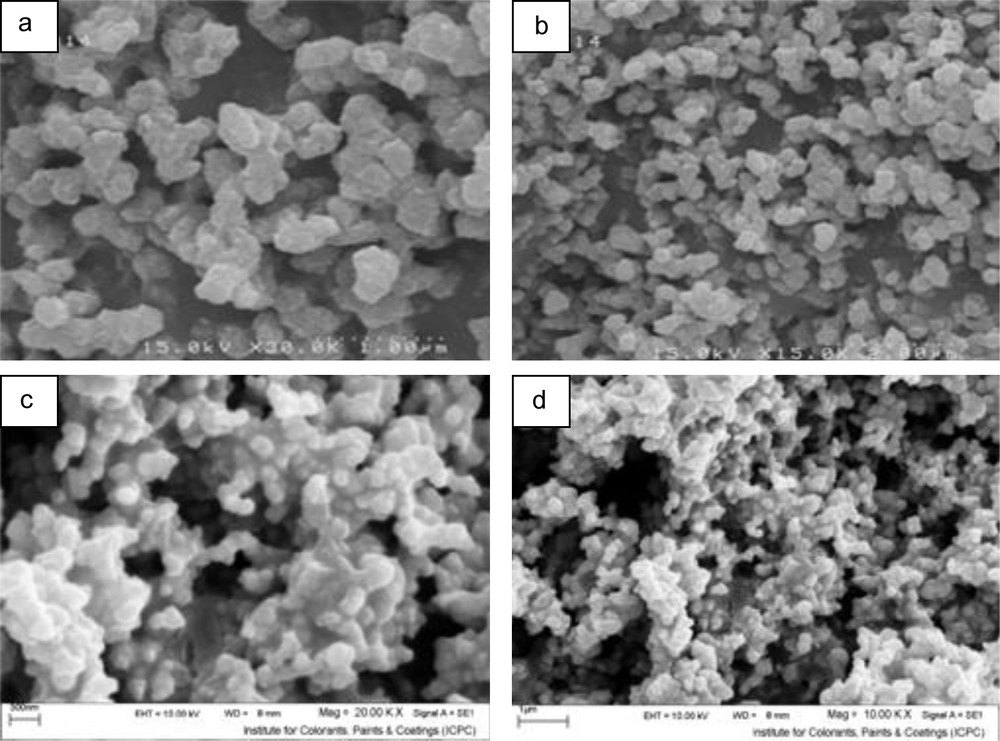
Scanning electron microscopy images of the product prepared using Ni(HAP)2 + thiocatamide with relation: (a) 1:1 (sample No. 2), (b) 1:2 (sample No. 3) and (c), (d) 1:4 (sample No. 4).

Scanning electron microscopy images at molar ratio 1:3, ethylene glycol solution (40 ml) at 600 W for: (a), (b) 5 min (sample No. 5), (c), (d) 10 min (sample No. 6) and (e), (f) 15 min (sample No. 7).
Fig. 9 shows the effect of different powers of microwave irradiation. It indicates that when microwave irradiation power changed, the size of the particles varied. The best power for this process was 600 W. During the process, the irradiation time was long enough to heat up the products to be at higher temperatures, which can lead to bigger sizes. At low power of microwave irradiation, there is not enough energy for the production of products and nucleation rate decreased. When microwave power increased from 300 to 600 W, the appropriate amount of energy was supplied in reaction medium, which affected nucleation rate and led to better nucleation. Further elevating of power led to the formation of many nuclei at the beginning of the reaction, which were very small and unstable. These small nuclei had a strong tendency for being stable, which led to agglomeration of products.
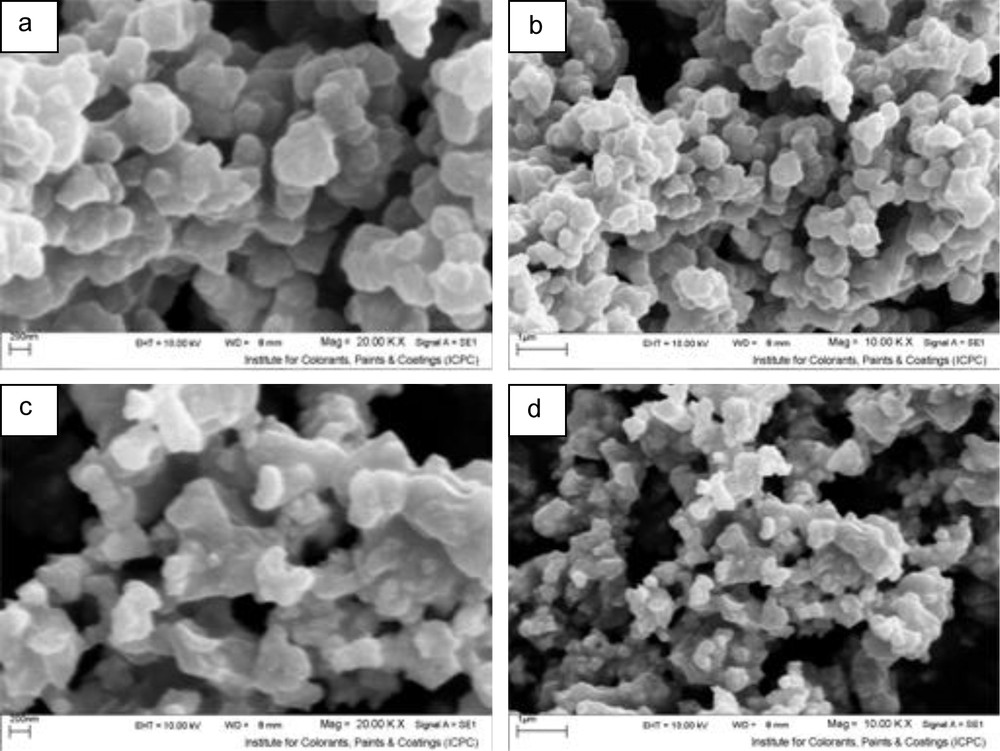
Scanning electron microscopy images at molar ratio 1:3, ethylene glycol solution (40 ml), 2.5 min inserted by microwave at: (a, b) 300 W (sample No. 8) and (c, d) 900 W (sample No. 9).
The effect of different solvent medium and precursors was also investigated. It was observed that when polyethylene glycol and water were used as solvent and TU and Na2S2O3 were used as sulfur source, no nanoparticles were evidenced. Also, the same result was obtained when Ni(OAC)2 was used as source of Ni ions (Fig. 10).
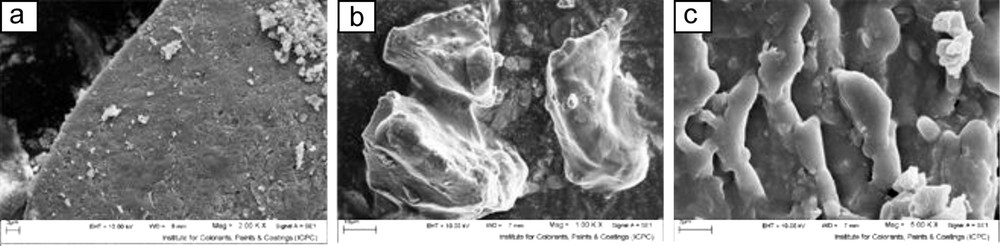
Scanning electron microscopy images at molar ratio 1:3, polyethylene glycol solution (40 ml), 2.5 min inserted by microwave at 600 W: (a) Ni(OAC)2 metal source, (b) Na2S2O3 sulfur source (sample No. 13) and (c) thiourea (sample No. 12).
4 Conclusion
NiS nanoparticles were successfully produced by microwave process at different conditions. This method is simple, energy efficient and shortens the reaction time. Furthermore, it is easy to continuously synthesize large quantities of products by using microwave equipment, which is significant for obtaining nickel sulfides in large amounts in industry. Different morphologies were characterized using SEM. The effect of different conditions, such as concentration of sulfur source, time of reaction, solvents, and power of microwave were investigated.
Acknowledgments
Authors are grateful to the council of Iran National Science Foundation and University of Kashan for supporting this work by Grant No (159271/55).