1 Introduction
Drug delivery is an interdisciplinary area of research in which people from almost every scientific discipline can make a significant contribution. Catanionic vesicles have already been applied as templates for drug-delivery applications as well as in nano-drug carrier synthesis [1–5]. Catanionic vesicles can be tailored in size and bi-layer thickness by changing the characteristics of the two surfactants, such as the type of the polar head and chain length, presence of salt and cholesterol, etc [6]. Owing to the vesicle structure, they compartmentalize the aqueous domain in the inner core of the vesicles which is separated from the outer water by a hydrophobic bi-layer [7]. They display therefore, interesting features like pH sensitivity as far as the synthesis of different stable vesicles of finite size is involved [8]. Greater the sensitivity of the vesicle architecture in differing pH in the local environment greater is the use as the template for drug delivery systems. However, as technology has advanced over the past few decades, methods of drug delivery became more sophisticated and rational, and people incite new strategies, including novel liposomes or vesicles for drug administration.
Attempts have been made to explore the potential applications of the vesicular systems to act as drug carriers in pharmaceutical industries. The formation, stability and biocompatibility studies of the mixed surfactant vesicles were thus examined in terms of different characterization techniques, hemolysis and MTT (3-(4,5 dimethyl-2-thiazolyl)-2,5-diphenyl-2H-tetrazolium bromide) assay. Although this type of mixed surfactant vesicle applied here exhibits enhanced stability in the presence of external stimuli, like salt, cholesterol, pH it is expected to encapsulate drugs in its core [6]. The drugs encapsulated in the aqueous core of the vesicles are also stable over a certain period of time in the physiological pH-temperature ranges, and can be considered as an effective drug delivery vehicle. Therefore, the main objective of the present study is to investigate the vesicle stability, pH-induced release of model drugs, hemocompatibility and cytotoxicity of the mixed surfactant vesicles using amino acid based carboxylate surfactants sodium N-alkanoyl-l-sarcosinate (SOS, SDS, SLS, and STS) of varying chain length and two commercial cationic surfactants cetyltrimethylammonium hydroxide (CTAOH) and dodecyltrimethylammonium hydroxide (DTAOH).
2 Experimental section
2.1 Materials
Sodium N-alkanoyl-l-sarcosinate (SAS) surfactants with different chain lengths (see Fig. 1 for structure) employed in this work were synthesized according to the reported methods [9,10]. l-alanine was obtained from SRL (Mumbai, India) and was used without further purification. The cationic surfactants, CTAOH and DTAOH, were prepared from CTAB and DTAB, respectively, by using anionic ion exchanger resin, where the ion exchange capacity of the anionic exchanger was fixed at ≥0.9 mM/ml. The model drug calcein (a fluorescent dye) was purchased from Aldrich (Milwaukee, WI, USA) and was used after recrystallization at least three times from an ethanol or acetone–ethanol (1:1) mixture. All the reagents and solvents used in this experiment were of good quality commercially available and were purified or distilled fresh whenever required.

Chemical structure of sodium N-alkanoyl-l-sarcosinate (SAS).
2.2 General instrumentation and solution preparation
All the measurements were carried out at room temperature (30 °C) unless otherwise mentioned. Temperature controlled measurements were carried out using a circulating water bath. Samples were prepared by combining the cationic and anionic surfactants at a desired concentration and mixing ratio from the individual surfactant stock solution. After sealing the samples were mixed by simple hand agitation. The composition of the mixtures thus obtained is expressed in terms of molar fraction, X1 (=[SAS]/([SAS] + [CTAOH])) of SAS. The pH of the samples was constant (pH 7.4) at 20 mM phosphate buffer having an ionic strength of 0.0126 M. Prior to observation and measurements, the solutions were allowed to equilibrate at room temperature for a 2–3 h. Transmission electron microscopy (TEM), dye entrapment and vesicle stability determination, hemolytic assay and cytotoxicity assay (cell culture and MTT assay) were performed as per our previous investigations [11].
The phase behavior of the SAS-CTAOH (or DTAOH) mixtures at different mixing ratios and concentrations was first investigated for aqueous solubility. It was observed that binary mixtures of SAS and CTAOH produced optically isotropic clear solutions at all compositions and total concentrations tested.
3 Results and discussion
The catanionic mixture formed by anionic amino acid based carboxylate surfactants sodium N-alkanoyl-l-alaninate (SOS, SDS, SLS, and STS) of varying chain length and two commercial cationic surfactants DTAOH or CTAOH with different chain lengths forms a strong synergistic interaction and stable vesicles in different compositions as discussed earlier [12]. The presence of vesicles are mostly seen at X1 = 0.50, whereas in other compositions both micelles and vesicles are found. The surface tension (also cmc) value of the mixed system is found to be minimum at different compositions and much lower than the pure and individual surfactants [13]. The fluorescence anisotropy values are also much higher (>0.140) in different compositions [11] which suggest the formation of vesicular systems which is further supported by the transmission electron micrograph (TEM) in Fig. 2. The TEM images show the hollow sphere structure of the vesicles and the hydrodynamic diameter (dH) of the vesicles is in the range of ∼30–200 nm. The drug molecules can easily be encapsulated in the aqueous core of these vesicles. The mixed surfactant vesicles are also stable (stability > 60 days), which can be used as drug carriers in pharmaceutical industry. The vesicles are also stable with time in the presence of additives like sodium chloride, cholesterol etc.
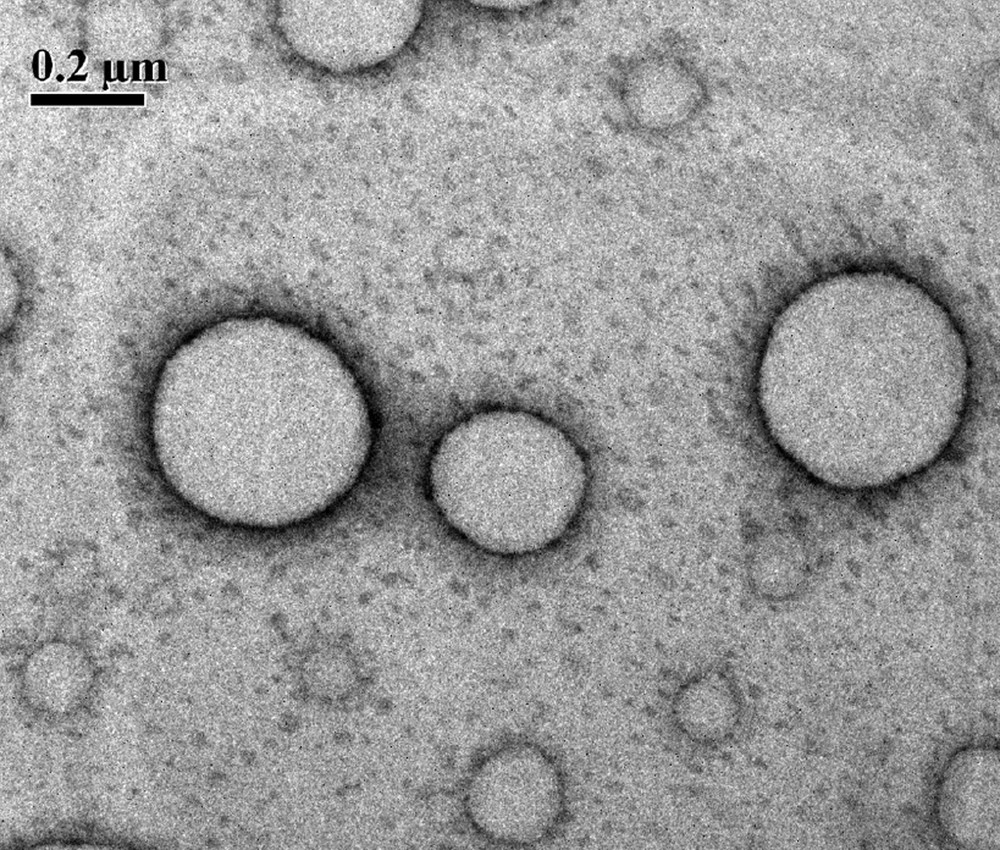
Negatively strained (1% uranyl acetate) transmission electron micrograph of the SLS-CTAOH (1 mM, X1 = 0.2) mixture.
In order to investigate drug entrapment ability and pH-induced release by the vesicle structures, calcein was used as a model drug. The pH-sensitive dye calcein was chosen as its four carboxylic groups get deprotonated at high pH. The tetra anion due to its low permeability remains entrapped inside the vesicles during these measurements. Consequently, fluorescence intensity of calcein decreases with the decrease of solution pH and also with time. Fluorescence spectra (Fig. 3(A)) of entrapped calcein (λex = 495 nm and λem = 520 nm) were measured immediately after dilution of the vesicle phase in a cationic-rich (X1 = 0.20) mixture of the SLS-CTAOH system in buffered solution of desired pH. Due to the decrease in solution pH vesicles are disrupt and calcein is released as shown by the quenching of fluorescence intensity (Fig. 3(A)). The entrapment and slow release of water-soluble drugs are thus demonstrated by the vesicles in the SLS-CTAOH mixture.
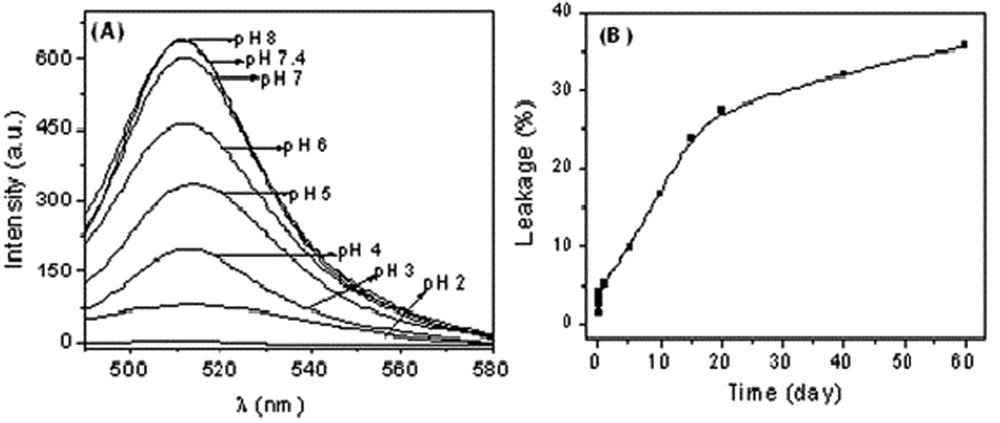
(A) Fluorescence spectra of vesicle-entrapped calcein in 10 mM SLS-CTAOH (X1 = 0.20) containing 10 mol % cholesterol at different pH values; (B) plot of extent of calcein released from SLS-CTAOH (X1 = 0.20) mixed vesicles at pH 7.4 as a function of time.
The long term stability of the dye-encapsulated vesicles was also considered. As time progresses, it is expected that the encapsulated dye would leak through the vesicle bilayer into the bulk solution. Due to self-quenching the fluorescence intensity of vesicle-entrapped dyes is very weak. However, the release of calcein from the vesicle core will enhance fluorescence intensity. The time-dependent increase of the fluorescence emission intensity of calcein was taken as a direct measure of the calcein efflux rates from vesicles. This is illustrated in Fig. 3(B), which shows the time trace obtained over the course of two months. Finally, the disruption was observed to be completed with the addition of Triton X-100, a nonionic detergent that disrupts both lipid and surfactant vesicles. Fig. 3(B) shows the % leakage of entrapped calcein from the vesicles formed by the cationic-rich SLS-CTAOH mixture (X1 = 0.20). It is seen that only 30–35% of the entrapped dye molecules were released during the period of observation. The relatively slow release suggests that the dye or drug entrapment and its subsequent release are not influenced largely with time and can be easily applied to the pharmaceutical industry.
In order to investigate the bioavailability of vesicles as vehicles in drug delivery applications, hemolytic activity was determined. The hemolytic activity is assessed by determining the hemoglobin release during the process of destruction of hematid. The results described later suggest that the vesicles can be efficiently used as a vehicle as they are found to be hemocompatible and do not show any hemolysis. However, before using in vivo, it is very much desirable to explore the compatibility of the surfactants with blood components. To evaluate this, hemolytic assays were performed for the three mixed surfactant systems SOS-CTAOH, SDS-CTAOH, SLS-CTAOH, and STS-CTAOH in two different compositions (X1 = 0.2 and 0.8) at pH 7.4 (HEPES buffer, 20 mM). Freshly isolated human red blood cell (RBC) suspension (5% v/v) was added to HEPES-buffered saline, 1% Triton X-100 and surfactants with a final concentration of 5 mM, and incubated for 60 min at 37 °C. The hemolytic activity (in %) of the different surfactant systems along with the +Ve and –Ve control are presented in Fig. 4. As seen, clearly none of the samples exhibits any hemolysis. It is known that any samples with less than 5% hemolysis ratio are nontoxic [14]. In the present investigation, it is observed that the surfactants SOS-CTAOH, SDS-CTAOH, SLS-CTAOH, and STS-CTAOH (X1 = 0.20) at a concentration of 5 mM exhibit 2.8 ± 0.11, 2.2 ± 0.05, 1.9 ± 0.11, and 1.5 ± 0.12% hemolysis, respectively. Therefore, surfactant mixtures containing different amino acid based anionic surfactants did not exhibit any significant lysis to the RBC membrane and hemolytic activity of the surfactants was hardly dependent on the chain length of the anionic surfactant. When the surfactant composition was changed to X1=0.8 the hemolysis was found to decrease to 2.3 ± 0.08, 1.9 ± 0.18, 1.6 ± 0.13, and 1.3 ± 0.11%, respectively. However, the values (<5%) still remain within the limit of toxicity. As seen, clearly none of the samples exhibits any hemolysis in comparison to –Ve control, that is, the surfactant mixtures containing amino acid-based anionic surfactants did not exhibit any significant lysis to the RBC membrane. Thus it can be concluded that the mixed surfactant vesicles can be efficiently used as a vehicle as they are found to be hemocompatible and do not give any hemolysis.
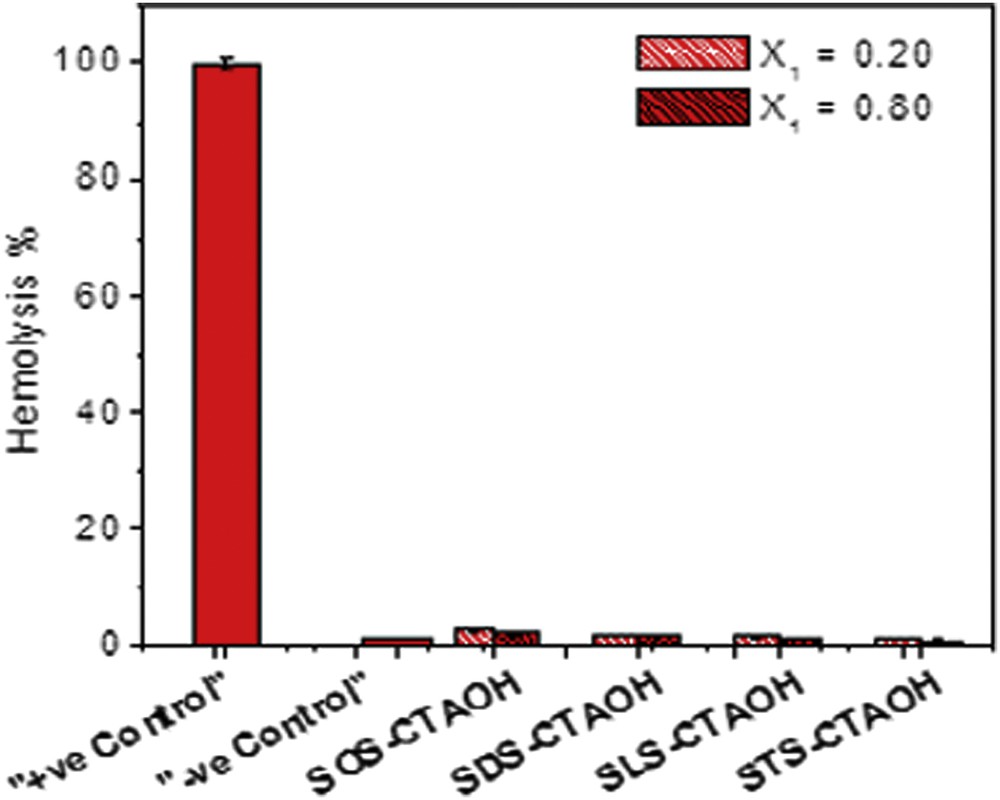
The plot of % Hemolysis of the 5 mM mixed surfactants at X1 = 0.20 and 0.80 at physiological pH (7.4). The bars indicate the means ± SD (n = 3).
The biocompatibility of the surfactant mixtures was further evaluated by measuring the cell viability against human cells. The cytotoxicity of the surfactants (5 mM) in two different compositions (X1 = 0.2 and 0.8) at physiological pH (7.4) was evaluated in order to identify their potential use in drug delivery applications. Cell viability was determined by measuring the viability of 3T3 cells using the MTT assay, based on the uptake and reduction of the soluble yellow MTT tetrazolium salt by mitrochondrial dehydrogenases to a blue insoluble MTT formazan product. The cell viability is shown in Fig. 5. No significant toxicity can be observed even at different surfactant compositions and more than 85% cells are still found viable after 48 h of treatment. To quantify the hemolytic effect of each treatment, the percent cell lysis relative to the untreated cell (% control) was determined by measuring the absorbance (540 nm) of the supernatant solution. Hence, the biocompatibility of the presently investigated surfactant systems was judged to be excellent. They are compatible to blood component RBCs and do not affect the cell growth and therefore can be used in pharmaceutical applications like drug carriers.
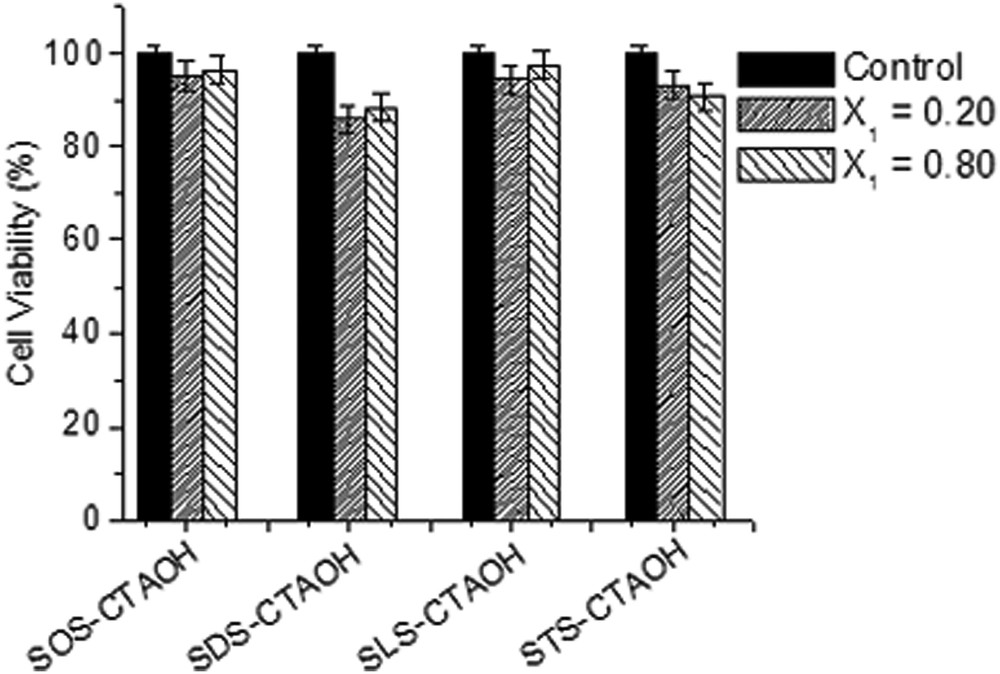
MTT assay based fibroblast cell line 3T3 cell viability (in %) as a function of surfactant composition (X1 = 0.20 and 0.80) at physiological pH (7.4). The bars indicate the means ± SD (n = 3).
4 Conclusions
These unilamellar catanionic vesicles formed by the binary mixtures of amino acid-based surfactants with CTAOH (or DTAOH) have hydrodynamic diameter in the range of 30–200 nm and drug entrapment ability of the vesicles suggest their stability at room temperature for a longer period of time. However, with the decrease in pH (pH ≤ 5) the mixed surfactant vesicles are transformed into small mixed micelles. This means that the vesicles are sensitive to pH change of the environment. These pH-sensitive vesicles are potentially interesting as drug delivery systems in which one aims to trigger the drug release from the vesicles as the pH is decreased from a neutral or slightly alkaline pH to an acidic pH. Bioavailability of the vesicles was determined to consider the formulation of a new encapsulating system as a vehicle in drug delivery applications. The biocompatibility studies, namely cytotoxicity and hemolytic potential, of the spontaneously formed pH-sensitive mixed surfactant vesicles have been evaluated. The mixed surfactant vesicles were found to be hemocompatible and did not show any hemolysis (<5%) against red blood cells. MTT assay also did not suggest any significant toxicity for any of the surfactant mixtures and ∼90% cells were found viable even after 48 h of treatment. The catanionic vesicles, because of their small size, stability, and nontoxicity to the blood component RBCs as well as mammalian cells can find applications in pH-triggered drug delivery. In fact, entrapment of water-soluble dyes into the vesicle core and its subsequent release triggered by pH change has been demonstrated in this work.
Acknowledgments
Authors gratefully acknowledge Central Research Facility, IIT Kharagpur, India for instrumental assistance.