1 Introduction
Mixtures of surfactants show enhanced performance in technical processes (e.g. detergency, tertiary oil recovery, drug carrier systems, flotation), when compared to pure surfactant systems. Surfactant mixtures for specific applications are often chosen based on empirical evidence and experience. However, to optimize the applications it is important to have a general understanding of the interplay of interactions between the surfactants in a mixed system and of the factors influencing the phase diagram. Therefore, it is of interest to study the self-aggregation and micellization of such mixtures.
When oppositely charged surfactants are mixed, new properties appear. Aqueous catanionic mixtures exhibit a wide range of unique properties that arise from the strong electrostatic interactions between the oppositely charged heads. They exhibit low critical micelle concentration (cmc) values and a non-monotonic change in the surfactant packing parameter (P) as the mixing ratio is varied [1]. For this reason a large number of aggregate structures such as spherical and rod-like micelles, vesicles, lamellar phases, and precipitates have all been observed depending on the concentrations, the size of the chain length or nature of the polar heads, and the ratios of the surfactants in solutions [2–9]. One advantage of catanionic systems as compared with more robust genuinely double-chained surfactants is their greater sensitivity to parameters such as temperature [10] or the presence of salts [11].
The effect of salt type on various physico-chemical properties of a system was first observed over 100 years ago by Hofmeister, who discovered the dependence of protein solubility on the type of added inorganic electrolyte [12]. Since then, a wide range of ion-specific phenomena in biology, pharmacy, and chemistry were observed. They have been recently reviewed in a special issue of “Current Opinion in Colloid and Interface Science” [13]. Extensive studies have shown that the counterion has a strong effect on the thermodynamics and aggregation properties of surfactants [14–16]. Salt effects on the cmc, micellar size, and degree of dissociation for a given surfactant may follow a lyotropic (or Hofmeister) series [17]. An ion's position in the lyotropic series can be correlated with its charge and hydrated radius. Depending on the charge density of the anion, it can interact more or less strongly with the cationic headgroups of micellar surface. Such a “binding” decreases the electrostatic repulsion between the surfactant headgroups and hence favors aggregation. This lowers for instance the cmc and the degree of ionization of the micelles. A typical Hofmeister series for anions is as follows: SO42− < C2H3O2− < Cl− < NO3− < Br− < ClO4− < I− < CNS− (the positions of the NO3− and Br− ions are often switched in the lytropic series).
Single-tailed surfactants usually form globular micelles in aqueous solution above their cmc [18]. An increase in surfactant concentration may induce the formation of worm-like micelles [19,20]. Similarly, addition of organic and inorganic counterions [19–22], uncharged compounds like aromatic hydrocarbons [23], or an oppositely charged surfactant [4,24] can transform spherical micelles into worm-like micelles.
Recently, we have proposed possible explanations for the different behavior of alkylcarboxylates and alkylsulfates with respect to counterions [25]. The arguments were strengthened by MD simulations [26]. We also were able to show that salt-induced micelle-to-vesicle transitions in the anionic-rich regions of the phase diagrams in catanionic systems depend on both ion and headgroup specificities [25,26]. In the present manuscript, this study is extended to the cationic-rich region. We focus on the influence of the counterion identity on the aggregation behavior of non-equimolar mixed surfactant solutions, composed of sodium dodecanoate (SL) and dodecyltrimethylammonium bromide (DTAB) with an excess of DTAB. The effect of different anions and cations on the micellar solutions will be shown by means of phase diagrams, cryo-TEM, mass spectrometry and dynamic light scattering. Collins' concept of matching water affinities [27] and recent work on ion specificity [26] will be shown to be also very valuable for the comprehension of the found series of salt sensitivity. And finally, the aggregation behavior will be compared to the one found in the anionic-rich region of the corresponding phase diagram.
2 Experimental section
2.1 Materials
The surfactants, sodium dodecanoate (SL; Sigma, Germany; grade: 99–100%) and dodecyltrimethylammonium bromide (DTAB; Merck, Germany; assay >99%) were used as received. All sodium and chloride salts used in the experiments were supplied by Merck, Germany. They were also used as received without further purification. Millipore water was used as solvent in all cases.
2.2 Phase diagrams
Surfactant stock solutions were prepared by dissolving weighed amounts of dried substances in Millipore water. The solutions were then left for 24 h to equilibrate at 25 °C. The catanionic solutions were prepared by mixing the surfactant stock solutions to obtain a fixed anionic/cationic surfactant mass ratio of 30/70. The starting ratio was determined from phase diagrams. The total surfactant concentration was kept at 1 wt.% at all times. The solutions then contain 14 mM of SL and 23 mM of DTAB. Salts were added to the micellar solution at increasing concentrations. The solutions were then stirred and left to equilibrate for a day at 25 °C before making measurements.
2.3 Cryo-transmission electron microscopy (Cryo-TEM)
Specimens for cryo-TEM were prepared as described elsewhere [25]; samples were examined with a Zeiss EM922 EF Transmission Electron Microscope (Zeiss NTS mbH, Oberkochen, Germany).
2.4 Electrospray mass spectrometry (ES-MS)
Cation affinities for the vesicular interface/carboxylate group were determined by electrospray mass spectrometry. ES-MS was carried out using a Thermoquest Finnigan TSQ 7000 (San Jose, CA, USA) with a triple stage quadrupole mass spectrometer. The solutions were sprayed through a stainless steel capillary held at 4 kV, generating multiply charged ions. Data were collected using the Xcalibur software. The surfactant concentration was kept 1 wt.% and the concentration of the various sodium salts was 20 mM in all cases.
2.5 Dynamic light scattering (DLS) measurements
Particle size analysis was performed using a Zetasizer 3000 PCS (Malvern Instruments Ltd., England), equipped with a 5 mW helium neon laser with a wavelength output of 633 nm. The scattering angle was 90° and the intensity autocorrelation functions were analyzed using the CONTIN software. All measurements were performed at 25 °C.
3 Results and discussion
3.1 Ion binding in catanionic surfactant mixtures
In the presently studied catanionic system, the cationic surfactant DTAB is in excess. Therefore, it is expected that the variation of the anions will produce a larger effect as the variation of the type of cations. Fig. 1 shows electrospray ionization mass spectra of the SL/DTAB catanionic solutions (mass ratio 30/70) upon the addition of different salts. Although in this method the ionization process takes place in the gas phase, the chemical nature of surfactant monomers and of simple ions is the same as in the liquid. Therefore, counterion affinities for the surfactant can easily be noted [28,29]. Because our surfactant mixtures already contain counterions, we add comparable concentrations of salts (remember that the solutions contain 14 mM of SL and 23 mM of DTAB). In this way, we can observe the competition of the counterions for the surface of the surfactant aggregate.
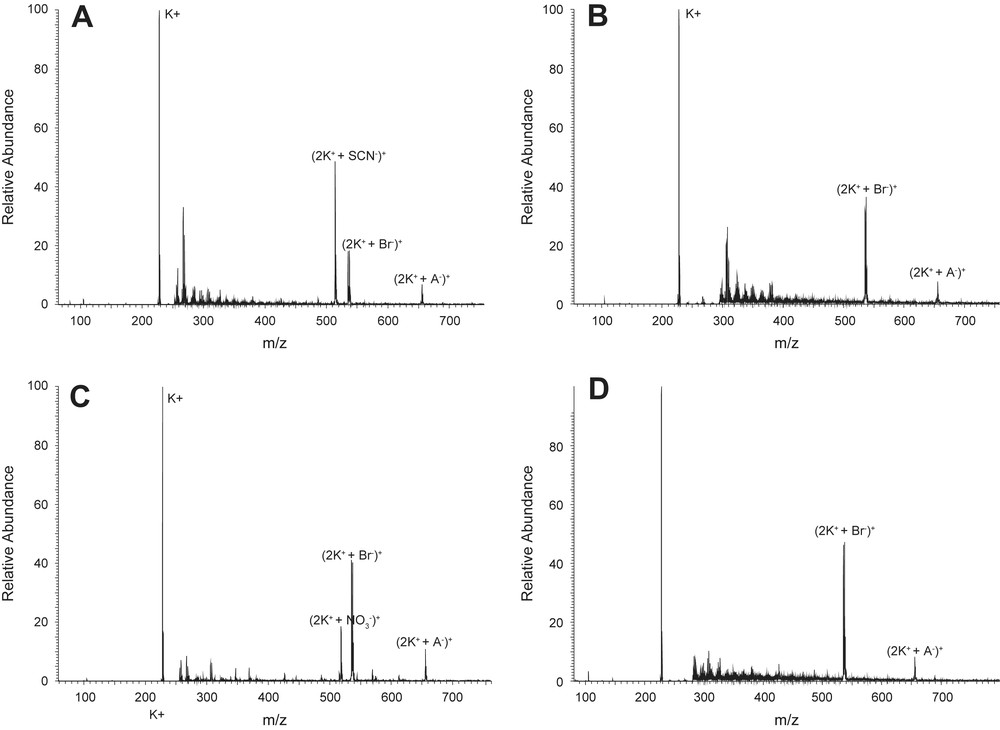
Ion binding as determined by ES-MS: addition of 15 mM of (A) NaSCN, (B) NaBr, (C) NaNO3 and (D) Na2SO4 to an SL/DTAB micellar solution. A−: dodecylcarboxylate anion (199 Da); K+: dodecyltrimethylammonium cation (228 Da); the peaks between 250 and 300 (m/z) are solvent related.
Fig. 1 represents the DTA+ fragmentation patterns upon the addition of various salts: for better visibility only a part of the m/z region is presented. It turns out that most of the mass signals (peaks) remain unchanged, independent of the nature of the added salts.
A closer look reveals that the position of the peak representing formation of ion pairs between the oppositely charged surfactant ions (2K+ + A−)+ remains unchanged. Also the size of the peak is comparable in all spectra. Similarly, the peak corresponding to the binding of bromide ions to the dodecyltrimethylammonium cation (2K+ + Br−)+ is present in all spectra, regardless of the nature of the added salt. The height of this peak, however, is less pronounced in spectum (A). When comparing the binding of anions to the dodecyltrimethylammonium cation, we observe that only SCN− exchanges the bromide anions at the micellar surface. NO3− and Cl− (c.f. Fig. S1, Supporting information) show approximately the same affinity for the alkyltrimethylammonium group, whereas in spectrum (D), when Na2SO4 is added to the catanionic mixture, no peak representing the binding of sulfate ions is visible. These results suggest that the strongly hydrated sulfate anion does not come close to the micellar surface, whereas the loosely hydrated thiocyanate anion is able to come closer to the micellar surface, replacing a part of the bromide ions at the interface. A general ordering of the cations can be determined from the ES-MS spectra, with thiocyanate showing the greatest affinity for the anionic group and the other cations following: SCN− > Br− > NO3− > Cl− > CH3COO− > SO42−. Note that a strong dehydration of cationic headgroups by bromide ions was directly measured via a determination of the effective area per headgroup, in agreement with our findings [30].
The variation of the cation of the salt produced no effect on the cation fragmentation patterns, as can be expected in a system with an excess of cationic surfactant. The m/z peaks remained unchanged for all added chloride salts.
3.2 Phase behavior upon salt addition
The influence of salts on the SL/DTAB catanionic mixture was further followed by observing the phase behavior; the starting phase point in the phase diagram being a micellar solution, c.f. diagram shown in Fig. S2, Supporting information. Different salts affect the system in various ways. While the addition of some salts produces no visible effect, others induce an easily observable aggregation (formation of a bluish color or turbidity). As the salt concentration is increased the aggregation becomes more pronounced. The effects are reported in Table 1.
Visual observations of the effect of various salts on the SL/DTAB (mass ratio: 30/70) catanionic solution. The reference sample is a clear homogenous solution.
Salt | 25 mM | 50 mM |
NaSCN | Bluish/turbid | Turbid |
NaBr | Bluish/turbid | Turbid |
NaNO3 | Bluish | Turbid |
NaOAc | Clear | Clear |
Na2SO4 | Clear | Clear |
NaCl | Clear | Clear/bluish |
LiCl | Clear | Clear/bluish |
KCl | Clear | Clear/bluish |
CsCl | Clear | Clear/bluish |
Choline Cl | Clear | Clear |
The aggregation was checked by light scattering; however problems were reported by the apparatus due to the high scattering intensity and the high polydispersity of the solutions. Despite that we are able to confirm an increase in the hydrodynamic radius of the particles in accordance with our visual observations (c.f. Fig. S3, Supporting information). We emphasise that the absolute values of the hydrodynamic radii should be taken with a grain of salt, because the aggregates formed in solutions are rods (as will be shown with cryo-TEM further on), and the CONTIN software used by the Zetasizer 3000 calculates the radius of spherical aggregates. However, the trend in the increase of RH is important. The degree of micellar growth varied in exactly the inverse order of the hydrated radius of the counterion: SO42− ≈ CH3COO− < Cl− < NO3− < Br− < CNS−.
Salts containing big anions having a weakly distributed charge (SCN−) induce more efficiently the micellar growth than the divalent sulfate (SO42−). Apparently the large hydrated radius of the sulfate ion hinders its ability to bind to the micelle containing an excess of DTAB.
3.3 Anion specificity in physico-chemical properties of alkyltrimethylammonium systems
The competition of various anions for the binding sites on the surface of CTAB micelles was checked by Larsen and Magid [31]. They found a strong binding of nitrate to the micelle, displacing bromide. The positions of bromide and nitrate ions are often exchanged in the lyotropic series. As an example, Cohen and Vassiliades [32] found that NO3− was less effective than Br− at lowering the cmc of CTAB. The lyotropic series was observed also when micellization of alkyltrimethylammonium halides, heats of counterion binding, surface tension, and the thermodynamic and microenvironmental properties of the micelles were compared [31,33–36]. The controlling factor in this ion specificity seems to be the distance of closest approach of the ion to the micelle.
3.4 Influence of salt on the aggregation behavior of surfactants
The geometry of aggregates in colloidal systems is attributed to the packing of the amphiphilic molecules. The factors governing the shape of the aggregates are expressed in its simplest form by a packing parameter P = v/(lmax·a), which is dependent on the length (lmax) and volume (v) of the hydrophobic tail and the effective size of the hydrophilic headgroup (a). The area per molecule at the interface depends on the hydration of the surfactant headgroup, which in turn depends on the ion charge density and the distance to the small counterions. Furthermore, it can be influenced by the ionic strength of the solution. When ions come close to the headgroups, the charges are more or less neutralized, the value a (area per molecule at the interface) decreases, and consequently the structural packing parameter P increases [37]. As a result, a decrease of the critical micellar concentration and a change in the aggregate morphology can be observed [37–39].
As was mentioned above, the system under consideration has an excess of DTAB. Previous studies have shown that the self-aggregation behavior of alkyl tetramethylammonium surfactants is relatively independent of the hydrocarbon chain length [40]. The phase behavior of DTAB (C12) and CTAB (C16) is therefore comparable. The formation of rod-like micelles in CTAB systems at high concentrations is widely known [40–44]. Alkylammonium halides have also been reported to exhibit a transition from spherical to rod-like micelle shape with increasing concentration of added salt. At lower ionic strength, only spherical micelles are formed, while at salt concentrations higher than a certain threshold, larger rod-like micelles are formed in equilibrium with the spherical micelles. The length of the rod-like micelle increases strongly with increasing ionic strength concentration [20,45–56]. Ozeki and Ikeda [46] reported that the sphere–rod transitions of micelles took place in aqueous NaBr solutions of DTAB when the salt concentration exceeded 1.8 M, whereas measurements of Sudan Red B in the same system indicated that the minimum NaBr concentration required to induce the sphere–rod transition was 1.0–1.5 M. This may be due to a decrease in intramicellar repulsion by electrical shielding due to ionic atmosphere. At higher salt concentrations, there is a dehydration of the spherical micelles due to the salting-out effect of NaBr.
By adding an anionic surfactant, we lower the charge density of the previously cationic micelles. In this way, we also lower the concentration of salt necessary to produce cylindrical micelles. Similar effects are observed with the addition of anionic hydrotropes to cationic surfactants [57–59]. Hydrotropes bind strongly to oppositely charged surfactant ions and reduce the headgroup area of the surfactant by reducing the headgroup repulsions. Thus, they are effective at promoting the elongated micelle formation.
The elongation of cylindrical micelles was studied by cryo-transmission electron microscopy. Fig. 2 shows that our initial micellar solution (reference sample) exhibits the presence of spherical micelles, as well as some short rods. An elongation of the cylindrical micelles is observed as salts are added to the solution. These micelles are quite flexible; small loops are seen, and the long micelles form networks. This causes an increase in the viscosity (observed at the preparation of the solutions) and an increase of the turbidity of the solutions. However, the growth and the concentration increase of the rods are salt specific (Fig. 3). The addition of NaBr produces a higher concentration of longer rods than the addition of NaNO3. These then start networking together and forming a net-like structure.
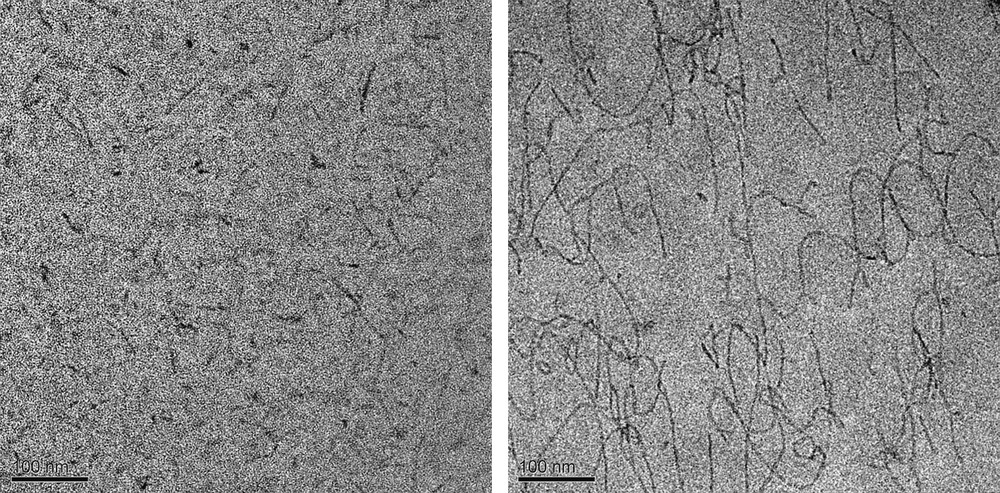
Left: spherical and small rod-like micelles in the SL/DTAB reference solution (before the salt was added); right: significant growth and networking of rods upon the addition of salt (25 mM NaBr).
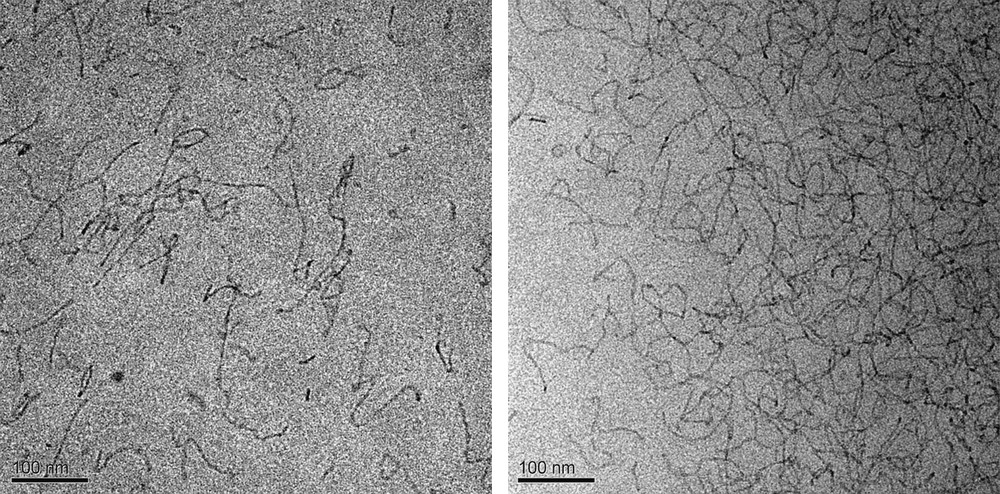
Cryo-TEM images representing the cylindrical growth as a function of anion type; the effect of 25 mM NaNO3 (left) and NaBr (right).
Surprisingly, we cannot see any formation of vesicles. Upon the addition of salts, the system goes from spherical micelles and small rods to long cylindrical micelles, and eventually to precipitation as the salt concentration is increased further. On the other hand, we have observed the transition from rod-like micelles to vesicles in the anionic-rich region of the phase diagram of this sample (when SL was in excess). A similar system to ours was studied by Sierra et al. [60]. They investigated the phase behavior of catanionic mixtures composed of DTAB and sodium undecanoate. They found the presence of spherical micelles on the anionic-rich side of the phase diagrams and short rods on the cationic-rich side. The rods elongate and aggregate as the composition nears equimolarity. No hexagonal mesophase was detected and the phase separation at equimolarity is preceded by the formation of bundles of rod-like micelles. The entanglement of long thread-like micelles in CTAB micelles upon salt addition was observed also by Aswal et al. [61].
3.5 Explaining counterion specificity in surfactant systems
Since the cationic surfactant is in excess in the catanionic system, and also in the mixed micelles, it is reasonable to suppose that anions are in average closer to the micellar surface than cations. Consequently, the effect of anion variation is more pronounced than the effect of cation variation. But what makes the difference between anions of the same charge? Is it their charge density and polarizability, in line with the ordering from hard (high charge density) ions, such as acetate, to soft (low charge density and often highly polarizable) ions, such as thiocyanate?
In a very interesting paper, Collins recently established a concept of so-called “matching water affinities”. According to this idea, soft ions should come in close contact with soft ions and hard ions in close contact with hard ions [27]. By contrast, when hard and soft ions come together, they do not approach so far that they lose their hydration sphere. Therefore, the interaction between a hard and a soft ion should be weak in water. Numerous phenomena in physical chemistry can be explained by this concept.
Recently, we could extend the usual Hofmeister series to surfactant headgroups. Jungwirth and his group used molecular dynamics (MD) simulation to classify them from hard to soft, in the same spirit as the ions [26]. For quaternary ammonium ions, it is not surprising that they behave like soft, low-density ions. According to Collins' concept it is therefore clear that DTA+ interacts more with softer ions than with harder ones, and this is precisely what is found in the present study. For example, SCN− ions come in close contact to the cationic headgroup and they share a common hydration shell, whereas acetate anions stay away from the headgroup and keep their own hydration shell. Consequently, the packing parameter will increase more, when NaSCN is added to the micellar solution than when NaOAc is added, and therefore the tendency to form rod-like micelles is more pronounced in the case of NaSCN.
3.6 Different self-aggregation behavior of catanionic systems in the catanionic- and anionic-rich regions
Why we are able to get vesicles in the anionic-rich region, but only thread-like micelles in the catanionic-rich region is a difficult question. This phenomenon was already observed by other authors, but never really explained. If the changes in the packing parameter are considered, the values of the hydrophobic part remain the same, only the ‘a’ value differs. However, the values for the parameter a (as reported in literature, e.g. Sierra et al. [60]) do not differ enough to explain this significant difference in the end packing. Nevertheless, even small differences in the headgroup area may lead to local segregation of anionic and cationic surfactants, as found by Dubois et al. [62]. One of the best explanations is the different hydration behavior of the headgroups compared to the hydration of the counterions. With the help of dielectric relaxation spectroscopy Buchner et al. were able to observe that the micelle surface of the SDS surfactant is strongly hydrophilic and the adsorbed counterions are generally separated by a layer of water molecules [63]. On the other hand, the surface of the alkyltrimethylammonium bromides is more hydrophobic, with the bound halide ions directly attached. It would be worth looking deeper into this asymmetry between DTAB and SDS. But this is beyond the scope of the present paper.
4 Conclusion
A study of the influence of added salt on the aggregation behavior of non-equimolar mixed surfactant solutions was conducted. The system was composed of sodium dodecanoate (SL) and dodecyltrimethylammonium bromide (DTAB) with an excess of cationic surfactant. The addition of salts produced a spherocylindrical growth of the micelles, markedly dependent on the anion identity. The efficiency of the anions to elongate the micelles could be explained by Collins' concept of matching water affinities and the classification of the cationic surfactant headgroup as a soft, polarizable entity.
Acknowledgements
This paper is dedicated to Pierre-Gilles de Gennes, one of the most eminent scientists of the last decades. W.K. is particularly indebted to him, as Prof. de Gennes was one of the reviewers of his “Habilitation à diriger des recherches” in France. The authors also thank J. Kiermaier, University of Regensburg for performing ES-MS measurements. M.D. gratefully acknowledges financial support by the Deutsche Forschungsgemeinschaft (SFB 481). N.V. is grateful to the DAAD for the financial support.