1 Introduction
Lately, an intensification of the research concerning solid base catalysts compared to those dedicated to acid catalysts has been noticed. An explanation of this trend is that the catalytic activity of solid bases was less explored in fundamental research and their utilization instead of soluble bases that are used in homogeneous catalytic processes may bring important benefits by overcoming the environmental issues related to the corrosive effect of caustic solutions. Alkali oxides, mixed oxides, basic resins, basic zeolites, and alkali metals supported on different supports were among the investigated systems. Besides those, the layered double hydroxides (LDHs) discovered in the 19th century can be substituted with good results for corrosive base homogeneous catalysts [1–4]. The general chemical formula is [M2+1−xM3+x(OH)2]x+[An−x/n]·mH2O, where M2+ and M3+ represent divalent and trivalent cations in the brucite-type layers, A is the interlayer anion with charge n, which neutralizes the excess positive charge occurring due to isomorphic substitution of M2+ with M3+, x is the fraction of the trivalent cation (x is usually 0.20–0.33) and m is water of crystallization [1]. These materials belong to anionic clays [5–8], rarely encountered in nature compared to the cationic clays. Hydrotalcite (HT) with the chemical formula Mg6Al2(OH)16CO3·4H2O is a naturally occurring solid belonging to the LDH class [1], having R3m space symmetry. This solid can also be easily obtained by the coprecipitation method either at low supersaturation [1,9–11] or at high supersaturation [12,13]. The products obtained by coprecipitation at low supersaturation are usually more crystalline compared to those prepared at high supersaturation conditions [14]. Other routes for preparing HTs are (1) the “urea” method [15,16], where Mg(NO3)2·6H2O and Al(NO3)3·9H2O are mixed with urea; (2) the hydrothermal method [17,18] consisting of physically mixing MgO and Al2O3 oxides in an aqueous suspension under a thermal treatment for different extents of time; (3) sol–gel synthesis [19], where a solution of ethanol and HCl is stirred with magnesium ethoxide and aluminum acetylacetonate; and (4) the mechanochemical method [20–22], which involves the grinding of solid salts in an agate mortar.
Throughout the time, the HTs and mixed oxides have found application in various fine chemical synthesis reactions such as aldol condensation [23,24], Knoevenagel reaction [25], Michael addition [26], cyanoethylation [27,28], Baeyer-Villiger oxidation [29], mild oxidation of S-containing organic compounds [30], and so forth.
Cyanoethylation (the basic catalyzed addition of compounds with labile hydrogen atoms such as alcohols, amines, and so forth to acrylonitrile) of alcohols is an important reaction to obtain alkoxypropionitriles, propanamines, propionic acids, important products for the syntheses of drug intermediates, and fine chemicals [31]. This reaction resembles closely to the Michael addition, which also requires the presence of base catalysts, but it is limited by the base-catalyzed polymerization of acrylonitrile [32]. Therefore, the catalysts used must be highly selective to the main product and not for the side reaction of polymerization. According to Figueras et al. [28], Tichit et al. [27], Chaphekar and Samant [33], and Hattori et al. [34], the catalytic activities for cyanoethylation depend on the type of alcohols and the type of base catalysts used. Also, it has been found that the adsorption of CO2 at room temperature does not have a poisoning effect because the alcohols are strongly adsorbed on the base sites as compared to carbon dioxide [35]. In what concerns the solid base catalysts, the basicity of HTs, mixed oxides derived from HTs by calcination, and the corresponding reconstructed LDH obtained by the memory effect differ in strength and number of base sites. Some of our recent studies showed that the modification of HT with low amounts of yttrium as in Mg3Al2.96Y0.04 leads to an increased basicity of the LDH solid due to the lower electronegativity value of the modifier as compared to magnesium and aluminum from the HT structure. However, the value of a parameter in the X-ray diffraction (XRD) analysis did not vary, suggesting that yttrium did not substitute Mg and Al from the brucite-type layer [36]. A small increase in Y concentration as in Mg3Al2.95Y0.05 leads to an enhancement of a parameter, which suggested that some of Y was incorporated in the brucite-type layer [37].
To the best of our knowledge, the effect of the incorporation of a higher concentration of Y in LDHs was not investigated, neither the reconstruction ability of these modified LDHs. Usually the reconstruction is performed by immersion of the mixed oxide form in water or aqueous solutions at room temperature under CO2-free atmosphere, when it is aimed to avoid contamination with CO2 from air. However, we suppose that this type of contamination could also be avoided if the reconstruction is performed at higher temperature, when the solubility of CO2 in the aqueous environment is less significant. Therefore, we considered a matter of interest to study the effect of Y concentration on the synthesis of Y-modified LDHs and the effect of the temperature during the reconstruction of the derived mixed oxides. To this aim, Mg3AlxY(1−x)-LDHs (x = 0.04–0.6) were prepared. The fresh LDHs, the derived mixed oxides, and the reconstructed samples obtained by rehydration at 25 and 70 °C, respectively, were characterized by chemical analysis, XRD, DRIFTS, BET, and determination of base sites. The catalytic activity of these solids for cyanoethylation of ethanol with acrylonitrile was investigated. The results of characterization and catalytic activity tests were compared to those obtained for a standard HT structure.
2 Experimental section
2.1 Catalysts preparation
The standard HT Mg3Al-LDH was obtained by coprecipitation under low supersaturation conditions [38] at pH 10, by mixing at room temperature under vigorous stirring (600 rpm) a 1.5 M nitrate aqueous solution containing the appropriate amounts of Mg(NO3)2·6H2O and Al(NO3)3·9H2O with a basic solution containing NaOH and Na2CO3 (concentration 1 M Na2CO3) used for pH adjustment. The feed rate of the two solutions was adjusted using TIM854, NB pH/EP/Stat pH-STAT Titrator to maintain the constant pH 10 during the procedure. The obtained gel was aged for 18 h at 75 °C, cooled to room temperature, filtered, and washed with bidistilled water until the conductivity of the washing water was less than 100 μS/cm. The cake was dried at 90 °C for 24 h in air flow. The corresponding mixed oxide, Mg3Al-MO, was obtained by calcination of Mg3Al-LDH at 460 °C for 18 h in air flow. The reconstructed samples Mg3Al-LDHR25 and Mg3Al-LDHR70 were obtained by immersing Mg3Al-MO in bidistilled water at 25 °C and heated 70 °C, respectively, aiming to reconstruct the HT structure by means of the memory effect.
For the preparation of yttrium-modified samples the preparation respected the same protocol, mentioning that for the preparation of the 1.5 M nitrate aqueous solution, besides Mg(NO3)2·6H2O and Al(NO3)3·9H2O, the corresponding amount of Y(NO3)3·6H2O was added to respect the following compositions: Mg3Al0.96Y0.04; Mg3Al0.6Y0.4; Mg3Al0.5Y0.5; and Mg3Al0.4Y0.6. The LDH samples were abbreviated as Mg3Al0.96Y0.04-LDH, Mg3Al0.6Y0.4-LDH, Mg3Al0.5Y0.5-LDH, and Mg3Al0.4Y0.6-LDH. The mixed oxides were named as above changing LDH suffix with MO and LDHR25 or LDHR70, respectively, for hydrated samples.
2.2 Catalysts characterization
The catalysts were characterized by chemical and elemental analyses. The determination of the metal content was performed by atomic absorption spectrometry using a Varian AAS spectrometer. N, H, and C were determined by elemental analysis using a Carlo Erba automatic analyzer.
Powder XRD patterns were recorded with a Shimadzu XRD 7000 diffractometer using Cu Kα radiation (λ = 1.5418 Å, 40 kV, 40 mA) at a scanning speed of 0.10° min−1 in the 5°–80° 2θ range.
DRIFTS spectra obtained from accumulation of 400 scans in the domain 400–4000 cm−1 were recorded using a NICOLET 4700 spectrometer.
N2 adsorption–desorption isotherms were determined using a Micromeritics ASAP 2010 instrument. Before nitrogen adsorption, samples were out gassed under vacuum for 24 h.
The basic character of the catalysts was determined using a method based on the irreversible adsorption of organic acids of different pKa values corresponding to the total number of base sites, for example, acrylic acid, pKa = 4.2, and strong base sites, for example, phenol pKa = 9.9 [38–41]. The number of weak and medium base sites is given by the difference between the amounts of adsorbed acrylic acid and phenol.
2.3 Catalytic tests
Cyanoethylation of ethanol with acrylonitrile (Scheme 1) was performed in a glass batch reactor equipped with a condenser system where acrylonitrile and ethanol (C2H5OH/C3H3N = 3/1 molar ratio) were stirred and heated on a silicon oil bath to the reflux temperature. Once the reaction temperature was reached, the catalysts (concentration of 3% (w/w)) were added and the reaction started. To monitor the progress of the reaction, samples of 0.5 μL were periodically removed from the reactor, analyzed by the GC K072320 Termo-Quest Chromatograph equipped with an flame ionization detector (FID) and a capillary column of 30 m length with DB5 stationary phase. Highly pure N2 (99.999%) was used as carrier gas. The products were identified by comparison with a standard sample (retention time in GC). They also have been identified by mass spectrometer coupled chromatography, using a GC/MS/MS VARIAN SATURN 2100T equipped with a CP-SIL 8 CB Low Bleed/MS column of 30 m length and 0.25 mm diameter.
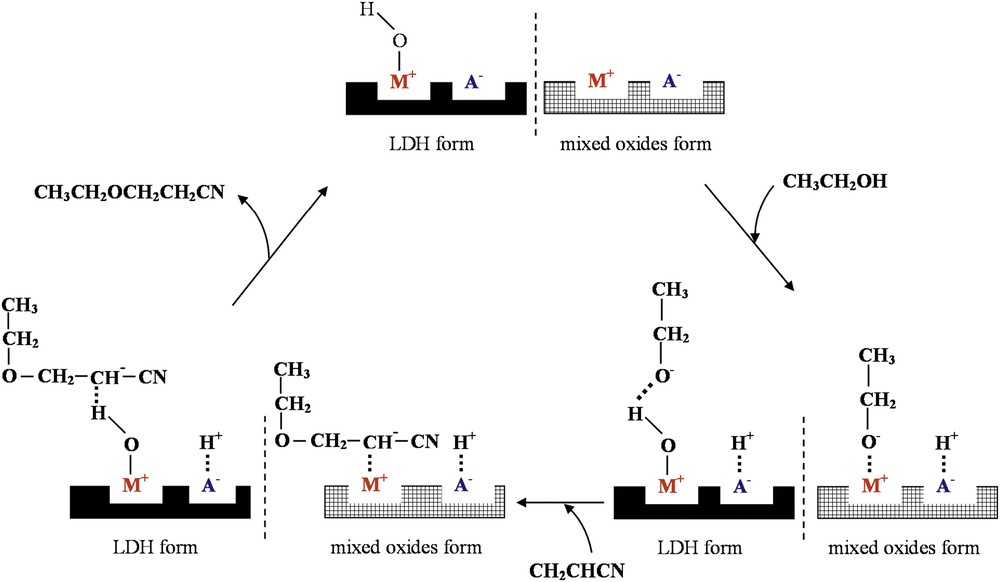
Reaction route of cyanoethylation of ethanol with acrylonitrile (M+ = Mg, Y, Al cations and A− = anion).
3 Results and discussion
3.1 Characterization
The results of the chemical analyses (Table 1) show that the addition of yttrium in the coprecipitation step led to an increase in the Mg/Al atomic ratio of the synthesized HT from 3.05 to 7.49. For medium and large amounts of yttrium, precipitation does not occur completely because there are very small amounts of nitrogen (0.01–0.02 wt %). For all samples (besides mixed oxides obtained by calcination), the quantity of carbon decreases for hydrated samples as compared to the corresponding parents because in the calcination step part of carbonate anions are removed. There is not a complete removal of carbonate anions because these are totally eliminated only above 600 °C, but at this temperature the memory effect disappears because the cations diffuse from octahedral positions in tetrahedral positions leading to the formation of a stable spinel [42]. The difference in terms of the carbon content when the hydration is made at different temperatures, 25 and 70 °C, is related to the fact that at 70 °C the solubility of the CO2 in water is lower, and therefore its intercalation would be less favored. Chemical analyses of the reconstructed samples showed that there is no significant difference between the amounts of hydrogen at 25 and 70 °C for the reference Mg3Al and the samples modified with the lowest quantity of yttrium Mg3Al2.96Y0.04. An increased amount of H appears for the samples with a higher amount of yttrium, for example, Mg3Al0.5Y0.5 and Mg3Al0.4Y0.6, when the reconstruction is performed at 70 °C, suggesting that the water amount embedded in these samples increases with the yttrium content. This is in agreement with the basicity values of samples.
Chemical/elemental analysis of samples, network parameter, and surface area of samples.
Sample | Mg/Al ratioc | Chemical composition (wt %) | a (Å) | c (Å) | Ssp (m2/g) | ||
N | C | H | |||||
Mg3Al-LDH | 3.05 | – | 2.08 | 4.18 | 3.062 | 23.314 | 128 |
Mg3Al-LDHR25 | 2.98 | – | 1.87 | 4.22 | 3.063 | 23.159 | 3.2 |
Mg3Al-LDHR70 | 2.99 | – | 1.76 | 4.23 | 3.059 | 22.998 | 8.5 |
Mg3Al0.96Y0.04-LDH | 3.13 | – | 2.08 | 4.17 | 3.070 | 23.880 | 123 |
Mg3Al0.96Y0.04-LDHR25 | 3.09 | – | 1.88 | 4.21 | 3.068 | 23.637 | 4.5 |
Mg3Al0.96Y0.04-LDHR70 | 3.10 | – | 1.75 | 4.23 | 3.064 | 23.528 | 11.3 |
Mg3Al0.6Y0.4-LDH | 5.5 | 0.02 | 4.59 | 4.12 | 3.078 | 22.242 | 118 |
Mg3Al0.6Y0.4-LDHR25 | 5.2 | 0.02 | 3.49 | 4.24 | 3.076 | 23.476 | 3 |
Mg3Al0.6Y0.4-LDHR70 | 5.2 | 0.02 | 3.14 | 4.36 | 3.074 | 23.572 | 8.1 |
Mg3Al0.5Y0.5-LDH | 6.04 | 0.01 | 4.60 | 4.10 | 3.086 | 23.252 | 102 |
Mg3Al0.5Y0.5-LDHR25a | 6.25 | 0.01 | 3.77 | 4.27 | 3.090 | 23.376 | 2.8 |
Mg3Al0.5Y0.5-LDHR70a | 6.26 | 0.01 | 3.25 | 4.47 | 3.082 | 23.976 | 6.2 |
Mg3Al0.4Y0.6-LDH | 7.49 | 0.02 | 4.62 | 4.13 | 3.098 | 23.565 | 87 |
Mg3Al0.4Y0.6-LDHR25a | 7.40 | 0.02 | 4.20 | 4.27 | 3.091 | 23.670 | 2.3 |
Mg3Al0.4Y0.6-LDHR70a | 7.41 | 0.02 | 3.55 | 4.49 | 3.096 | 23.774 | 4.5 |
Mg3Al-MOb | – | – | – | – | 4.199 | – | 188 |
Mg3Al0.96Y0.04-MOb | – | – | – | – | 4.210 | – | 175 |
Mg3Al0.6Y0.4-MOb | – | – | – | – | 4.223 | – | 164 |
Mg3Al0.5Y0.5-MOb | – | – | – | – | 4.224 | – | 145 |
Mg3Al0.4Y0.6-MOb | – | – | – | – | 4.228 | – | 138 |
a a and c values in italics for the samples showing a poor reconstruction.
b Samples of mixed oxides with cubic structure.
c As determined from the results of AAS analysis.
The XRD patterns (Fig. 1A) present the typical patterns of a pure LDH structure with sharp and symmetric reflections 003, 006, 110, and 113 and broad and asymmetric for 012, 015, and 018. Modification with a low amount of yttrium does not change the XRD intensities, whereas at high amounts of yttrium the reflections become irregular and less intense. No others diffraction lines are present in XRD patterns of yttrium-modified samples. For lower amount of yttrium Mg3Al2.96Y0.04 the parameter a is not significantly changed (Table 1) because the substitution of magnesium or aluminum from octahedral sites does not take place; only c parameter increases due to the presence of some amorphous yttrium compounds such as hydroxide or carbonate in the interlayer region. However, these XRD patterns do not exclude the presence of different yttrium species on the external surface.
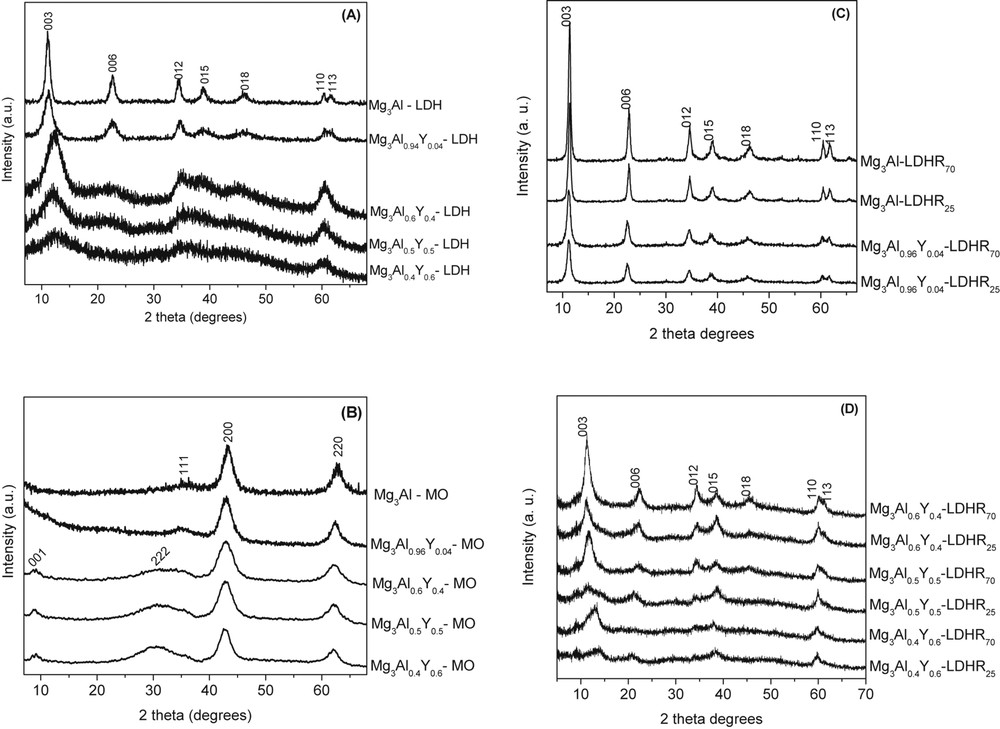
The XRD patterns of initial layered materials (A), mixed oxides obtained by calcination (B), and hydrated samples at different temperatures (C and D).
Although increasing Y content, the network parameter a for Mg3Al(1−x)Yx-LDH increases from 3.062 to 3.098 Å suggesting that there is an increased substitution of Mg/Al by the modifier cation. Therefore, it may be considered that the presence of the highest amount of Y is the driving force for obtaining a modified LDH structure, even if Y excess determines the formation of some other amorphous species. The XRD pattern of the calcined sample Mg3Al-MO (Fig. 1B) evidenced the formation of a MgO-periclase–like solid solution (JCPDS 45-0946), which is a defective structure with a certain degree of cation vacancies generated by the introduction of trivalent cations in the octahedral sites. For the mixed oxide samples Mg3Al0.6Y0.4, Mg3Al0.5Y0.5, and Mg3Al0.4Y0.6, the diffraction lines of yttrium oxide (Y2O3) have been detected for 001 and 222 reflections [43]. The singular oxidic phase of Y2O3 is probably generated from the calcination of some amorphous phases of yttrium hydroxide or carbonate, which were not detectable in the XRD of the parent LDH samples (see Fig. 1A). After hydration, by means of the memory effect, the HT-type structure is reconstructed for the reference Mg3Al and for the sample with the lowest amount of yttrium Mg3Al2.96Y0.04 (Fig. 1C), with the diffraction lines corresponding to 00l and 110 more intense than for the parent samples [37,38,44]. The amount of hydroxyl groups is higher than in the initial material because part of carbonate anions removed during calcination is replaced by OH− groups. This is in accord with the fact that c parameter after hydration decreases due to the lower radius of the hydroxyl group as compared to carbonate groups. The presence of yttrium (Fig. 1D) leads to a decrease in intensity of lines suggesting that yttrium oxides are not involved in the reconstruction of the LDH structure. For the pure HT and the sample modified with the lowest amount of yttrium the trend of variation for c parameter (Table 1) is LDH > LDHR25 > LDHR70, but for the samples with higher amount of modifier cation the variation is reverse. Therefore, it may be inferred that modification of HT with small amounts of yttrium does not lead to the modification of the structure.
The DRIFT spectra (Fig. 2) show that in all cases the vibrations assigned to OH and carbonate groups at 3700–3400 cm−1 corresponding to υO–H, 3070 cm−1 related to hydrogen bonding between water and carbonate in the interlayer of the LDHs, 1640–1650 cm−1 vibration of interlayer water, 1520 and 1400 cm−1 assigned to CO32− group vibration, and in the region 1200–700 cm−1 the bands characteristic to carbonates. The last one is present even for the mixed oxides indicating that carbonates cannot be completely removed even by thermal decomposition at 460 °C. The peak around 650 cm−1 in the DRIFT spectra of Mg3Al(1−x)Yx-LDH (Fig. 2A) is related to YOH stretching mode. After the calcination of LDH samples, new bands appeared at 570, 480, and 420 cm−1 characteristic to YO metal–oxygen stretching frequency in cubic Y2O3 (Fig. 2B). During calcination, part of OH− and CO32− are removed from the structure and the intensity of the signals at 3070 and 1640–1650 cm−1 decreased. After the hydration of the mixed oxides, the signals at 3070 and 1640–1650 cm−1 were restored. The band at 3704 cm−1 (Fig. 2C) corresponds to stretching vibrations of structural hydroxyl groups, which are coordinated to octahedral Mg species, and it appears only for the sample Mg3Al0.4Y0.6-LDHR70. This is in accord with Table 1 and with the fact that at higher quantity of yttrium the Mg/Al ratio increases and part of Mg is pushed outside of the HT structure as Mg(OH)2. For the same sample, Mg3Al0.4Y0.6-LDHR70, there is an increased intensity of the band around 1400 cm−1, which is shifted to lower wavenumbers as compared to the spectra of Mg3Al0.4Y0.6-LDHR25 and Mg3Al0.4Y0.6-LDH. This fact may be related to the presence of the external phase of Mg(OH)2, which can be carbonated. However, the presence of the bands in the region 600–400 cm−1 corresponding to the Mg–O, Al–O, and Y–O bonds may suggest that a part of mixed oxides was not reverted to the LDH form.
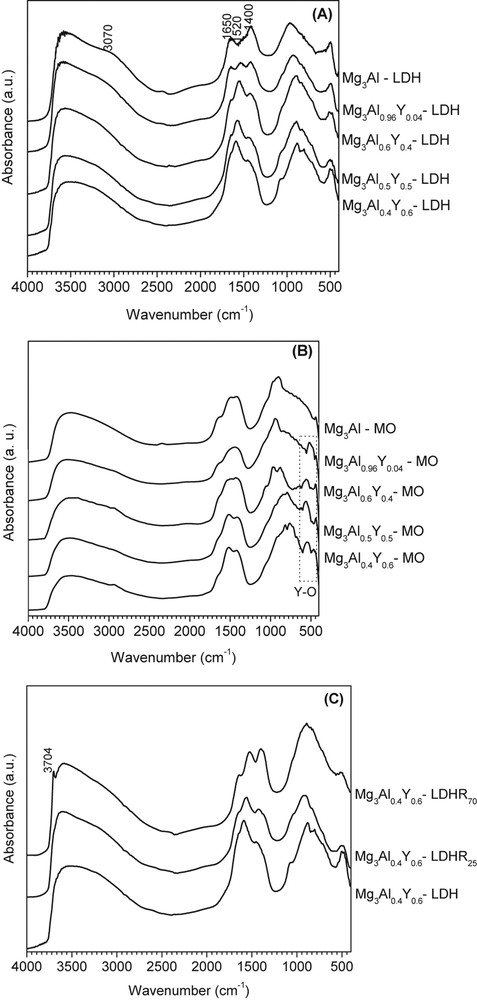
DRIFT spectra of the initial samples (A), mixed oxides obtained by calcination (B), and hydrated sample at 25 and 70 °C of Mg3Al0.4Y0.6 (C).
The surface areas for unmodified or modified samples vary as it follows: MO > LDH > LDHR70 > LDHR25 (Table 1).
The surface area of the Y-modified samples decreased from 123 to 87 m2/g, along with the increase in Y concentration. The specific surface areas of the mixed oxides are larger than those of the corresponding LDHs because of the removal of both water and CO2 from the interlayer region. All the samples were mesoporous with most pore diameters in the range of 50–150 Å. All the reconstructed samples had a lower surface area than the initial LDHs, a phenomenon that was first noticed for the reference sample, and it was explained by a twisting of the hexagonal plates in reconstructed LDH accompanied by the formation of vermiculate-shaped irregularities, which hinder the entrance of N2 molecules used in the determination of the specific surface area [37,42]. The reconstructed samples obtained by hydration at 25 °C had a lower surface area than those hydrated at 70 °C, which can be a consequence of an exfoliation effect favored by the higher temperature.
The values of the basicity (Table 2) show that the mixed oxides present a higher base character than HT or reconstructed LDHs for both temperatures due to strongest base character of O2− from the surface of samples. The presence of weak base sites is associated with the surface OH− groups, whereas the medium strength base sites are related to the oxygen bridging among Mg2+, Al3+, and Y3+ cations. The basicity of the LDH forms is lower because they present on the surface of both OH− and the CO32− groups. Because the number of CO32− is higher than OH− and because this anion possess a weak acid character the entire value of basicity in the case of LDH samples is low. Instead, after hydration of mixed oxides by partially replacing of CO32− with OH− anions an increase in the basicity occurs because the second anion has a stronger base character. The trend of the total basicity variation is the following: MO > LDHR70 > LDHR25 > LDH. Slightly increased basicity for hydrated samples at 70 °C as compared to 25 °C is due to the incorporation of a larger amount of OH− groups during hydration, a fact confirmed by elemental analysis results from Table 1. The yttrium-modified samples present a slightly increased basicity value as compared to the HT material because the Pauling electronegativity of this cation is lower, and therefore, the base character is stronger (Al3+ (1.61) and Y3+ (1.22)) [45]. The above presented basicity values include also the base sites due to the oxygen vacancies, which play the same role in the extraction of protons from the alcohol molecule as the electrons belonging to the superficial oxygen anions from the lattice. A limitation of the method used for the determination of base sites is that it does not allow to discern between oxygen vacancies and other types of base sites.
The basicity of samples determined by means of irreversible adsorption of organic acids of different pKa values and conversion values after 5 h.
Samples | Total base sites (10−3 mol AA/g) BT | Strong base sites (10−3 mol PhOH/g) BS | Weak and medium base sites BW = BT − BS | Conversion of acrylonitrilea (%) |
Mg3Al-LDH | 6.73 | 0.26 | 6.47 | 22.3 |
Mg3Al-LDHR25 | 7.21 | 0.34 | 6.87 | 38.1 |
Mg3Al-LDHR70 | 7.52 | 0.41 | 7.11 | 44.2 |
Mg3Al0.96Y0.04-LDH | 6.93 | 0.32 | 6.61 | 30.5 |
Mg3Al0.96Y0.04-LDHR25 | 7.62 | 0.38 | 7.24 | 48.2 |
Mg3Al0.96Y0.04-LDHR70 | 7.96 | 0.44 | 7.52 | 57.4 |
Mg3Al0.6Y0.4-LDH | 7.72 | 0.31 | 7.41 | 49.2 |
Mg3Al0.6Y0.4-LDHR25 | 7.78 | 0.42 | 7.46 | 53.7 |
Mg3Al0.6Y0.4-LDHR70 | 8.00 | 0.46 | 7.54 | 58.1 |
Mg3Al0.5Y0.5-LDH | 8.01 | 0.48 | 7.53 | 57.5 |
Mg3Al0.5Y0.5-LDHR25 | 8.12 | 0.50 | 7.62 | 62.4 |
Mg3Al0.5Y0.5-LDHR70 | 8.21 | 0.51 | 7.70 | 68.3 |
Mg3Al0.4Y0.6-LDH | 8.27 | 0.52 | 7.75 | 70.3 |
Mg3Al0.4Y0.6-LDHR25 | 8.37 | 0.55 | 7.82 | 75.2 |
Mg3Al0.4Y0.6-LDHR70 | 8.48 | 0.58 | 7.90 | 80.6 |
Mg3Al-MO | 8.36 | 0.38 | 7.98 | 87.2 |
Mg3Al0.96Y0.04-MO | 8.72 | 0.42 | 8.30 | 91.3 |
Mg3Al0.6Y0.4-MO | 9.11 | 0.51 | 8.60 | 94.1 |
Mg3Al0.5Y0.5-MO | 9.36 | 0.65 | 8.71 | 96.6 |
Mg3Al0.4Y0.6-MO | 9.50 | 0.68 | 8.82 | 98.4 |
a After 5 h of reaction time.
3.2 Catalytic tests
The ethanol/acrylonitrile molar ratio was chosen taking into account that the conversion increases by ca. 10% when the ratio increases from 1/1 to 3/1 (Fig. 3), whereas the further increase in this ratio up to 9/1 does not lead to increases in the conversion by more than 5%.
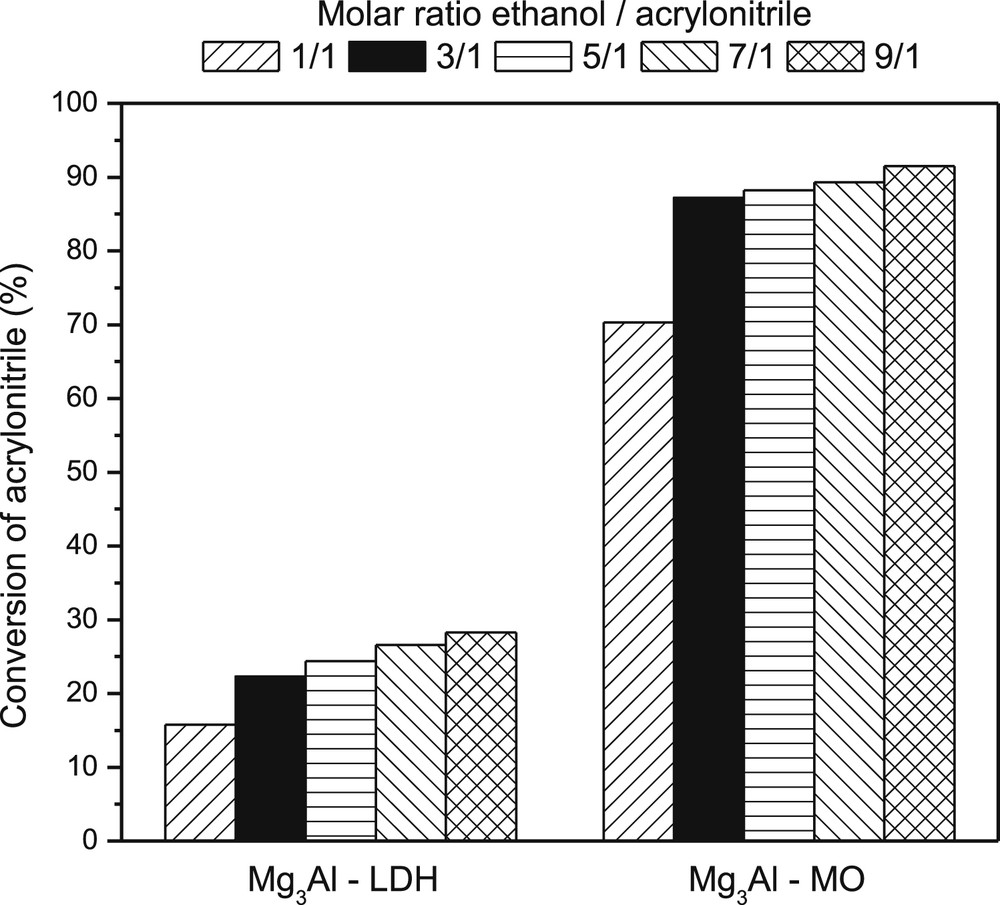
The activity values of Mg3Al-LDH and Mg3Al-MO samples in cyanoethylation of ethanol with acrylonitrile after 5 h versus ethanol/acrylonitrile molar ratio.
In addition reaction (Scheme 1), the samples show a remarkable selectivity of 99%–100% to β-ethoxyproprionitrile. For all initial samples the activity after 1 h of reaction time is low (Fig. 4A). At the highest amount of yttrium the conversion reaches about 20% rising to 70% after 5 h. The unmodified sample presents the lowest activity, whereas the modified samples show higher activity along with the increase in the yttrium amount. The modified samples display a special synergetic effect between the HT and yttrium hydroxides species.
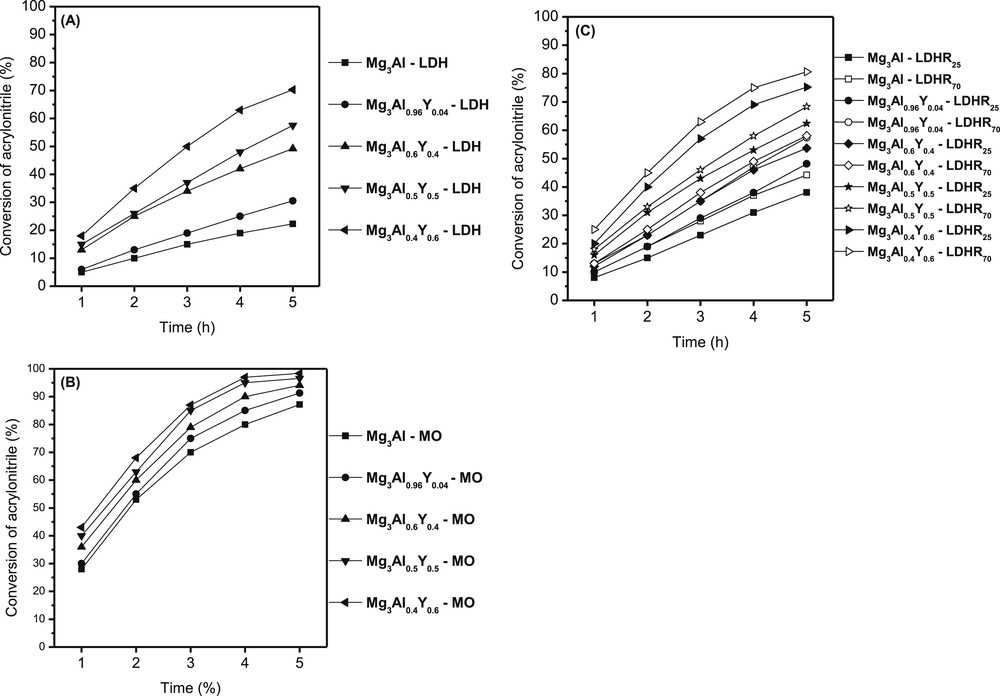
The activity values of samples in cyanoethylation of ethanol with acrylonitrile (initial layered materials (A), mixed oxides obtained by calcination (B) and hydrated samples at different temperature (C)).
Because after calcinations, the superficial O2− population is higher, the activities have the highest values. Even in this case, the most active sample is that with the highest amount of yttrium, which shows a conversion of 99% after 5 h. The higher number of OH− incorporated in the hydration step led to a good conversion for samples LDHR70 than LDHR25 as compared with initial ones. Because the number of OH− is higher in the samples hydrated at 70 °C, the conversion varies in the same way. Therefore, the variation in activity follows the order calcined samples (MO) > hydrated samples (LDHR70) > hydrated sample (LDHR25) > dried samples showing the important role of the base site strength.
A linear correlation between the acrylonitrile conversion after 5 h of reaction time and the total number of weak and medium strength base sites in the catalyst was observed (Fig. 5).
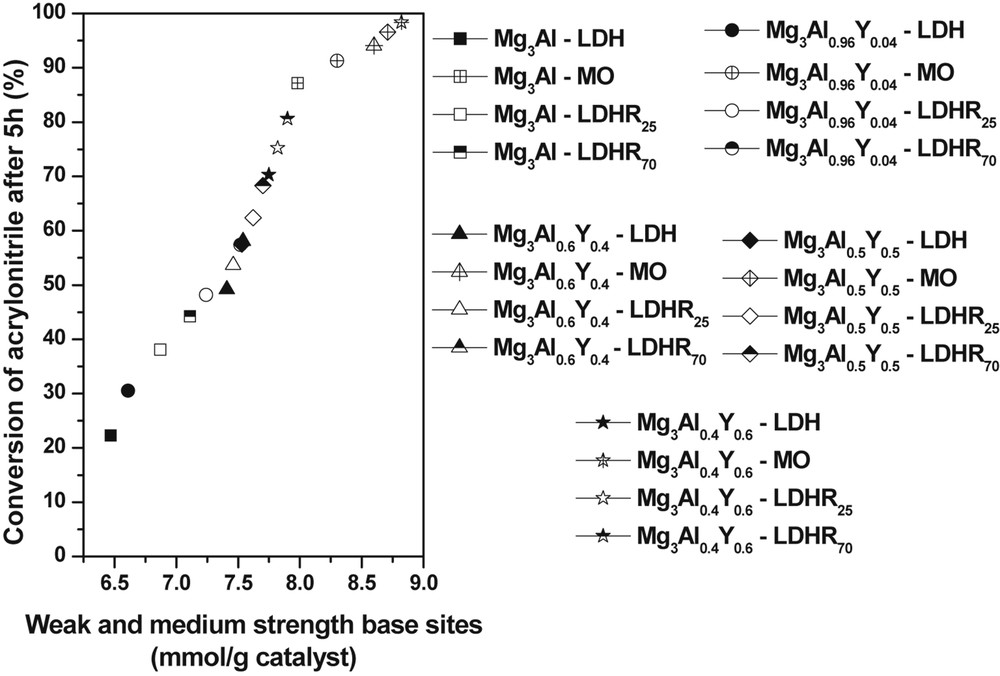
The linear variation of acrylonitrile conversion after 5 h of reaction time as a function of weak and medium strength base sites.
The absence of correlation between the total amount of base sites and the conversion of acrylonitrile after 5 h indicates that the strong base sites are not as reactive as the weak and medium strength base sites for this reaction. Therefore in agreement with previous findings [28,46–48], the activity in the cyanoethylation reaction depends more on the density of weak and medium strength base sites than on the total number of base sites.
In all cases the catalyst could be easily separated by simple filtration and reused. Induced coupled plasma analysis confirmed that no yttrium was present in the filtrate, showing no significant leaching. The most active catalyst was Mg3Al0.4Y0.6-MO. After performing five reaction cycles it has been noticed that the conversion of acrylonitrile decreases with only ∼2%–3%, whereas the selectivity of β-ethoxypropionitrile is still >99%.
4 Conclusions
The modification of HT with small amounts of yttrium in the preparation step does not bring a significant modification in the structure. The driving force for the inclusion of yttrium in the brucite-type layer is its presence in a molar amount exceeding aluminum. Hydration of mixed oxides obtained by calcination to reconstruct the initial structure can occur at different temperatures: 25 °C and 70 °C, respectively. At 70 °C the amount of OH− groups embedded is more pronounced than at a low temperature. The high number of OH− groups incorporated during reconstruction using the memory effect leads to the increase in the base character of samples, which is reflected in an increased catalytic activity. The increase in basicity is closely linked to the presence of yttrium due to its lower electronegativity. For all catalysts, the selectivity of β-ethoxyproprionitrile is more than 99% and there was no polymerization of acrylonitrile. The most active catalyst Mg3Al0.4Y0.6-MO was stable for more than five cycles.
Acknowledgments
This work was supported by a grant from the Romanian National Authority for Scientific Research, CNCS – UEFISCDI, project number PCCA 137/2012, HYLAYHY.