1 Introduction
Rivers represent the major link between continents and oceans in most biogeochemical cycles; therefore, riverine fluxes have been considered by scientists working on the Earth system as geochemists and biogeochemists since the first study of Clarke in 1924 [4,10,33]. For water managers, rivers are prime targets for direct water resource, and are also used for energy supply, commercial transportation. Yet the transformation of river systems by humans is now reaching a level at which this essential Earth system's component is controlled more by anthropogenic forcing than by natural drivers as climate, relief or lithology, at least where there are significant populations of humans [39,47,67,73]. This is a state that characterizes the Anthropocene era, as first postulated by Vernadski in 1926 [69]. This concept has been recently revived by Crutzen [12,13] mainly for the climate evolution, but it is also highly applicable to the evolution of river systems [42,44,73].
The global evolution of riverine fluxes during geological times has shaped the coastal zone, and fed the oceans with essential nutrients as phosphorus and silica [33,38], regulated long-term climate through CO2 uptake during continent weathering [3], and supporting biodiversity of continental aquatic systems [30]. The recent evolution of riverine fluxes over the last century is now beginning to impact the whole Earth system [53,65], yet this type of global change has so far not been expressively considered within the integrated water management concept advocated for a better use of the water resources [59,79], although it will be a central question of major Earth system's science initiatives [65], such as the newly consolidated Global Water System Program [18]. We address here five questions:
- (i) how variable were fluvial systems under natural conditions? – we consider here the last 18 000 years since the Last Glacial Maximum during which climate conditions have been extremely variable;
- (ii) how riverine fluxes are distributed over the land surface?
- (iii) what are the main factors controlling retention of river-borne material, namely ‘fluvial filters’ in addition to the well-recognized production factors such as lithology, runoff and relief?
- (iv) how have human activities modified the functioning of fluvial systems, particularly these fluvial filters, during the Anthropocene era?
- (v) can the global nature of land-to-ocean fluxes be captured by a single global trajectory, and how do regional differences manifest themselves and contribute to this pathway?
2 Databases and analytical tools
While the scientific community on global water issues is rapidly developing some critical global-scale resources [72,77], currently, global river databases are limited. We are using here a set of databases, many of them assembled within the IGPB-BAHC project and published for river runoff, reservoir location, river basin network and morphology, river sediment fluxes, present river chemistry [45,46,75,76,78] as well as other sources, such on river sediments [48] and on world river geochemistry [15]. A great number of river basin case studies have been used as well, a more detailed account can be found in [18,27,73]. For the past 18 000 years, we considered numerous studies and models as the Benito et al.'s synthesis [2] and other works that can be found in [24,73].
3 Natural Holocene changes of fluvial systems
Since the Late Glacial maximum (LGM), some 18 000 years ago, fluvial systems and land–ocean connections have been very dynamic [2,22,73] due to four major factors: (i) glaciation of some river basins, (ii) changes in river runoff due to the global climate change, (iii) varying exposure of new landmass due to eustatic lower sea level change and isostatic rebound, and (iv) distribution of continental area draining to the oceans (exorheism) or internal continental regions (endorheism) (Table 1A). During this period, climate is without doubt the driving force of the land–ocean connection as it controls river runoff, extent of ice cover and exposure of the continental platform.
Major changes of land–oceans connections since the Last Glacial Maximum (A) and during the Anthropocene (B)
Changements majeurs dans les connexions terre–océan depuis le dernier maximum glaciaire (A) et pendant l'Anthropocène (B)
A. Changes on continental area between 18 000 BP and Present (in Mkm2) Changements de surface continentale (en Mkm2) survenus entre 18 000 ans BP et l'Actuel | |||
18 000 BP area | Present-day area | Reference | |
Total ice-free | 129.9 | 133.2 | [22] |
Exposed shelf4 | 15.9 | 0 | [22] |
Additional land covered by ice4 | 18.4 | 0 | [22] |
Cumulated endorheic and arheic basins formerly linked to oceans1 | |||
10 to 15 | 0 | This work | |
B. Recent changes occurring mostly since 1950 (in Mkm2) Changements récents (en Mkm2) survenus, pour la plupart, depuis 1950 | |||
Total reservoir area | 1.1 | [62] | |
Total irrigated area | 2.5 | [62] | |
Total area intercepted by major reservoirs | > 40 | This work | |
Total area with more than 80% runoff reduction2 | > 4 | This work | |
Total area with more than 25% runoff reduction due to water use3 | |||
> 15 | This work |
1 Including Caspian and Aral Sea basins, Kerulen, Okawango, Mar Chiquita, Turkana and Chari Basins, parts of Niger Basin, Arabic and northern Sahara Basins.
2 Colorado (Arizona), Nile, Amu Darya, Syr Daria Basins.
3 The same plus most Mediterranean rivers, Rio Grande, Indus, Murray, Orange, Huang He, southern Soviet Union basins.
4 With regards to the present-day area.
At the LGM, most of North America, Europe and parts of Siberia were covered by ice from the Rockies to Labrador and from Norway to the Taimyr Peninsula. Meanwhile, the lower sea level uncovered huge continental platforms in eastern Siberia, Bering Sea and northern Canada, North Sea, the southern part of South China Sea, Arafura Sea, and Patagonia. These platforms were drained by fluvial systems much bigger than today: the paleo Malengraaf River draining Borneo, Java, Malaysia, Thailand, and the Mekong Basin [16], exceeded an area of , among the world's top ten basins.
The exact paleogeography of global river systems remains to be established [73]. In western Siberia, the paleo Ob was possibly blocked by the Scandinavia–Taimyr ice cap, thus forming a huge lake and swamp area of about [25]; however, the extent of this event is debated [34]. At that stage the blocked Ob waters could have flown south, through the Turgay River channel, to the Aral Sea, which was overflowing to the Caspian. The Northern Dvina, Kolyma and the Pechora were probably diverted and forced to flow backwards to the Caspian Sea or westwards to the Southern Baltic Sea. During the ice melt period, the level of the Caspian Sea was much higher and the Caspian was overflowing to the Black Sea through the Manych depression, forming a gigantic basin from central Asia to southeastern Europe.
More recently, climate variations have also generated important changes in the connectivity of fluvial systems: in Africa, the Okawango Delta and the Zambezi River were connected. In Asia, the Kerulen was linked with the Amur River and the Aral Sea was connected to the Caspian Sea through the Uzboi River on old maps. Other recent linkages have been found or are likely to have occurred on other continents (Mar del Plata system–Parana River; Chari/Chad system–Benue/Niger Basin; Turkana Lake/Sobat River-Nile). At earlier periods, the great basin in North America was also overflowing to the Columbia River through the Snake River. The exact timing of all these land–ocean connections remains to be established at the global scale.
During the wetter Sahara period, at 6000 BP, seasonal river runoff from the Air mountains to the Niger (Azawak River) and from the Hoggar and Tibesti mountains to the Lybian Gulf was likely [49,54]: huge basins as the ‘YeBaEg’ (area ) were very active but how much reached the Mediterranean sea or the Niger basin remains unknown. Some of the Nile tributaries, north of the 5th Cataract, and some of the river networks in the Arabic Peninsula probably were also active.
Few major river basins have been relatively stable since the LGM; they are essentially located in the wet tropics as the Amazon, Congo, Orinoco. Yet if their drainage area has not much changed, their average runoff may have fluctuated. Since these systems are among the most important suppliers of continental material to oceans, their flux variability will have to be taken into account in any global assessment. Examples of the past changes of some major riverine systems are presented in Table 2A.
Global changes on fluvial systems. (A) Compared evolution since the Last Glacial Maximum (LGM). (B) Natural fluvial filters (F1 to F5) under current climate conditions. (C) Artificialization of the waterscape by Humans and man-made filters (F6, F7). (F1 to F7: see Figs. 1 and 2)
Changements globaux dans les systèmes fluviaux. (A) Évolution comparée depuis le dernier maximum glaciaire (LGM). (B) Filtres fluviaux naturels (F1 à F5) dans les conditions climatiques normales. (C) Artificialisation du système hydrologique par l'homme (F6, F7). (F1 à F7 : voir Figs. 1 et 2)
(A) Past changes (last 18 000 yr) | (B) Natural filters, present conditions | (C) Waterscape artificialization | |||||||||||||
GL | Q | BA | A (*) | F1 | F2 | F3 | F4 | F5 | Fev | F6 | F7 | ||||
ARH | SLP | WTL | LK | FLP | EST | EVP | RES | IRR | DYK | DIV | |||||
Amazon | 6.1 | ++ | +++ | + | |||||||||||
Amur | + | +++(31) | 1.85 | + | + | ||||||||||
Bay James (32) | ++++ | (0.7) | +++ | + | + | +++(21) | +++ | ||||||||
Colorado | + | 0.64 | + | + | ++ | +++(19) | ++ | ++ | +++(20) | ||||||
Congo | 3.7 | + | +(38) | +++ | |||||||||||
Danube | + | 0.8 | + | ++ | ++ | ++ | + | ||||||||
Ganges/Brahmaputra | + | 1.63 | ++ | ++ | ++ | ||||||||||
Huang He | +++(27) | 0.75 | + | +++ (27) | ++ | +(18) | ++ | +++ | |||||||
Indus | + | 0.91 | ++ | ++ | ++ | ++ | +++(17) | +++ | |||||||
Irrawaddy | + | 0.41 | + | + | + | ||||||||||
Lena | + | 2.5 | ++ | +++ | |||||||||||
Mackenzie | ++++ | 1.8 | + | ++ | +++(3) | ++ | ++ | ||||||||
Mekong | + | ++++(26) | 0.79 | + | +(6) | +(6) | ++ | ++ | |||||||
Mississippi | + | 3.0 | + | + | ++ | ++ | ++(13) | ++ | |||||||
Murray/Darling | 1.0 | + | +++ | ++ | ++ | ||||||||||
Nelson | ++++ | 1.1 | ++ | +++(5) | + | ++ | +++(41) | ||||||||
Niger | ++(25) | 1.2 | ++ | ++(4) | + | ++ | + | ++ | + | ||||||
Nile | ++ | ++(24) | 2.9 | +++ | + | ++(1) | ++(2) | ++ | ++ | + | ++++(14) | +++ | |||
Ob | ++ | +++(23) | 3.0 | +++ | +(12) | ||||||||||
Orange | +++(35) | 1.0 | +++ | + | +++ | +++ | |||||||||
Parana | ++(33) | 2.8 | + | ++ | +++(34) | +++ | ++ | +++ | + | ||||||
Rio Grande | 0.9 | + | ++ | ++ | +++ | +++ | |||||||||
Sao Francisco | 0.63 | + | ++++ | ++ | ++ | ||||||||||
Shatt El Arab | 0.54 | ++ | +++ | +++(16) | ++ | + | |||||||||
St Lawrence | ++++ | 1.02 | + | +++(7) | |||||||||||
Yang Tsé Kiang (1) | + | 1.8 | + | +++ | +++(15) | ++ | ++(39) | ||||||||
Yenissei | ++ | + | 2.6 | + | + | ++(36) | ++ | + | ++ | ||||||
Yukon | 0.85 | ++ | + | ++ | |||||||||||
Zambeze | +++(29) | 1.3 | +(37) | + | +++(40) | + | ++(39) | ||||||||
Amu Darya # | + | ++ | ++++(28) | (0.4) | ++ | + | +++ | +++ | ++ | +++ | +++(22) | ||||
Syr Darya # | + | ++ | ++++(28) | (0.4) | + | + | +++ | ++ | +++ | +++ | |||||
Chari/Logone # | ++ | ++(?)(30) | (0.7) | + | ++ | ++ | + | + | |||||||
Eyre Basin # | ? | (>1.0) | +++ | + | + | +++ | |||||||||
Helmand # | ++ | ++(?)(24) | (0.4) | +++ | + | ++ | +++(10) | ||||||||
Kerulen # | ++ | ++++(31) | (1.0) | ++ | + | + | +++(11) | + | |||||||
Okawango # | ? | +++(29) | (1.0) | +++ | +++ | ++(8) | |||||||||
Tarim # | +++(24) | +++(24) | (0.5) | +++ | +++(9) | ++ | |||||||||
Volga # | +++ | ++ | 1.35 | + | ++ | ++ | +++(21) | ++ | ++ |
All these changes occurring on river basins in pristine conditions, prior to any human impacts over centuries to millennium, are likely to also generate temporal variabilities of river fluxes (sediments, major ions, nutrients, carbon).
4 ‘Hot spots’ of river fluxes to oceans in pristine conditions
Earth-system scientists need pristine fluxes for global models [65], established typically around the 1900s, when human pressures (deforestation, reservoir construction, flow diversion, pollution) were still limited [47]. This hypothetical pristine world is usually estimated from the present-day situation by subtracting all human impacts, and then the past fluxes are reconstructed with varying climate conditions. The future fluxes are generated with global change scenarios, including climate change and direct human pressures.
The combination of factors that control river-borne material production and mobilization (e.g., runoff, relief and lithology), and of retention factors (e.g., riparian vegetation, floodplains, natural lakes, deltas) results in a very skewed distribution of pristine river fluxes at the global scale. In semi-arid and arid areas, most of the continental surface is presently not actively connected to oceans, whereas in tropical wet environments it flows continuously. Since runoff is the number one factor of river fluxes [31,32,48], we have differentiated four categories of long-term annual runoff (q): (i) arheic , (ii) oligorheic , (iii) mesorheic , (iv) hyperheic for which the corresponding ionic fluxes have been estimated [43]. Due to interactions between the general atmospheric circulation, mountain belts, and the position of deep interiors of the continents, hyperheic regions are only found in the exorheic regions while the three others are shared between the exorheic and endorheic regions.
Long-term runoff is here based on global models constrained by field measurements collected by the global Runoff Data Center [19]. Earlier physical geographers and global hydrologists [14,28] were not able to distinguish some arheic regions, as Sahara, Saudi Arabia, parts of Kalahari, from true endorheic regions. Now a GIS analysis of global topography at a fine scale ( latitude x, longitude and less) can be performed: it has revealed that fossil river networks in these regions are potentially connected to oceans [76] and therefore both exorheic and presently arheic.
A first-order budget of land-to-ocean fluxes of major ions has been realized, differentiating exorheic and endorheic regions for the four runoff categories [43]. This budget shows that today, under the present pristine conditions (without any of the human perturbations presented further), the greatest part of the continents does not contribute to the major ions fluxes from land to oceans. These fluxes are currently dependent on the hyperheic regions ( continental area; 38% fluxes) and on the mesorheic regions of the exorheic realm ( area; 55% fluxes). These proportions are of the same order for all major ions including bicarbonates, i.e. dissolved inorganic carbon: 90% of the weathering of continents and its related land-to-oceans fluxes of carbon, nitrogen, phosphorous or silica is nowadays occurring on 30% of the planet, corresponding to about 90% of related fluxes.
This estimate has been realized on the basis of 685 medium-sized pristine rivers between and , for which the present distribution of ionic yields per square kilometer at the Earth's surface ranges over two to three orders of magnitude [43]. A similar range is also observed for the sediment yields, which may vary from less than 10 to about [48,81,82]. There is no doubt that such distributions would have been more skewed if established at a finer resolution. The distribution of riverine fluxes at the fine scale () is therefore extremely heterogeneous. A fraction of the continents, less than 10%, is probably contributing to the major part of river fluxes, with yields exceeding 10 times the global average, which can be used as a definition of a hot spot. For a comprehensive global analysis, these ‘hot spots’ of riverine fluxes will have to be identified and mapped for each river material.
The suspended matter budget has been reviewed by Milliman and Syvitski [48] on the basis of hundreds of river basins of various sizes. They clearly identified South and East Asia as the major sources of sediments to oceans and linked these hot spots to tectonically active continental margins in South Alaska, Taiwan, New Zealand [31,48].
This heterogeneity of riverine fluxes is still visible at the continental scales. Estimates of pre-industrial total nitrogen fluxes (Table 3) show marked differences in average yields. It is due to the balance between the productions factors, as lithology, relief and, most of all, runoff, and the retention factors that characterize fluvial filters. Production factors have been mostly addressed by geochemists [4,23,31,32,43,46,73], while retention factors have been considered by geomorphologists and biogeochemists [22,36,37,66,78,80–82]. We are focusing in the next sections first on natural filters, then on the present-day situation of fluvial filters with man-made filters and altered natural filters.
Evolution of total nitrogen specific fluxes (gN ) at the surface of continents (exorheic area). Recalculated from [23]. Note the inverted trends in Africa due to reservoir retention and irrigation
Évolution des flux spécifiques d'azote total (gN ) à la surface des continents (aire exoréique). Recalculé à partir de [23]. À noter les tendances inversées en Afrique par suite de la rétention des réservoirs et de l'irrigation
Continent | Pre industrial | Contemporary (1980–2000) | Tot N trend D/B ratio | ||||
A: Total N supply | B: River export | B/A ratio % | C: Total N supply | D: River export | D/C ratio | ||
Africa | 1.35 | 0.12 | 0.09 | 1.55 | 0.09 | 0.06 | 0.75 |
Asia | 0.85 | 0.22 | 0.26 | 2.6 | 0.55 | 0.21 | 2.5 |
Australasia⁎ | 1.17 | 0.073 | 0.06 | 1.3 | 0.1 | 0.08 | 1.4 |
Europe | 0.55 | 0.15 | 0.26 | 3.2 | 0.76 | 0.24 | 5.1 |
N. America | 0.50 | 0.12 | 0.24 | 1.3 | 0.20 | 0.16 | 1.65 |
S. America | 1.32 | 0.36 | 0.27 | 1.7 | 0.43 | 0.26 | 1.2 |
Global exorheic | |||||||
0.96 | 0.18 | 0.19 | 1.92 | 0.35 | 0.18 | 2 |
⁎ Australia and New Guinea.
5 Fluvial filters in natural systems
River basins are highly dynamic and cannot be considered as systems that totally and continuously transfer river-borne material. River material is actually recycled, stored, remobilized and transformed, while being transferred downstream at timescales ranging from less than one year to more than 106 years. These processes are well known for the river particulates [36,80] and can also be described through the Trimble's Diagram [37,66] expressing sources and sinks in a river basin.
Vegetal biomass, soils and their associated phreatic groundwater can be considered as a first omnipresent filter (Fig. 1, F0) that retains, transforms and transfers materials, originating from the atmosphere (fallout, CO2 uptake and N2 fixation) from soil chemical weathering and leaching or from the mechanical erosion of soils and rocks. Carbon and some key nutrients as potassium, nitrogen, phosphorus [58] and even silica [11] can be stored in the soil/vegetation filter. Once mobilized by headwaters, the fluvial material faces multiple filters that, in turn, can modify the riverine fluxes. Slopes and piedmonts (F1) are trapping the coarser particulates. Headwater wetlands (F2), abundant in low relief regions, are storing or transforming atmospheric inputs. Lakes (F3) are near-perfect traps for particulates and efficient biogeochemical reactors, like the seasonally inundated river floodplains (F4). Finally most estuarine types (F5), as macrotidal estuaries, coastal lagoons, deltas, deep fjords are characterized by important sedimentation rates and/or biogeochemical cycling [5,51] that again modify the net river fluxes to the coastal zone.
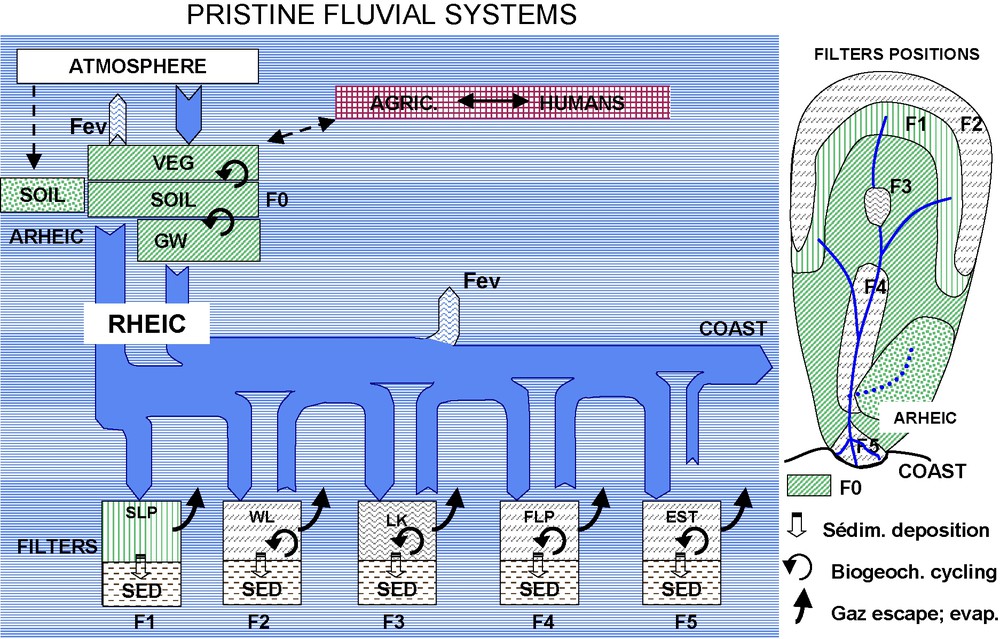
Schematic position of natural filters within pristine river systems. In addition to the soil/plant filter (F0), different types of filters control the pathways and transfers of river-borne material: slopes and piedmonts (F1), headwaters wetlands (F2), lakes (F3), inundated floodplain (F4), and estuaries (F5). Human impacts are very limited.
Positions schématiques des filtres fluviaux naturels dans les systèmes aquatiques continentaux. Différents types de filtres contrôlent les transferts de matériaux des continents aux océans via les rivières : la végétation et le sol (F0), les pentes et les piémonts (F1), les zones humides de têtes de bassins (F2), les lacs (F3), les plaines d'inondation (F4) et les deltas et les estuaires (F5).
These successive filters can be very efficient. In large river systems, the retention of particulates is around 90% with regard to the amount of material generated by local relief denudation [81] and this retention exceeds 99% when very large lakes are in residence (e.g., Baikal, Victoria, North America Great Lakes). Other river-borne material like organic carbon [63,64] and nitrogen [23] are also transformed into gaseous forms during their transit under natural conditions in fluvial systems.
The absence of flow (arheism) has been conventionally set here at , in arid regions where rainfall is very rare and does not generate any appreciable runoff, through a model of global interannual runoff on simulated topological river network at the resolution of 30 min (STN-30). In natural conditions, this threshold corresponds to about one flood event every 10 years. For exorheic rivers flowing through dry land (allogenic rivers) as for the Nile, this evaporative process can also be considered as a filter (Fev).
A tentative rating of major natural fluvial filters is presented on Table 2B for some of the largest river basins. This first attempt shows major contrasts among systems dominated by one type of filter as floodplain (as Congo and Yangtze Kiang), lakes (Saint Lawrence, Nelson), evaporative filter (Tarim, Kerulen), headwaters wetlands (Bay James Rivers).
Few systems show a predominant absence of natural filters like the Irrawaddy or Colorado, they generally correspond to convex average longitudinal profiles characterized by deeply incised valleys or canyons [75]. Slope deposition and floodplain retention are typical of highly concave profiles as for the Indus, Ganges-Brahmaputra, Orinoco and Amazon Rivers [75]. Mixed systems that combine multiple climate, relief and/or lithology characteristics, as the Nile, Mackenzie and Amazon, also combine multiple classes of natural filters (Table 2B).
The next step for global analysis will be to map and quantify these filters and their functioning, taking into account their extension and their position within each fluvial system. For example, lake filters may be quite different in their impact whether they are located in headwaters or close to the river mouth. The residence time of water in each system appears to be a key parameter in filtering efficiency for sediments [78] and, when modulated by temperature for regulating biogeochemical cycling, for total nitrogen retention [23].
6 Anthropocene modifications of fluvial systems
At the Anthropocene, land use and water use as well as wastes inputs or leaks generated by human pressures are deeply changing the production of river-borne material and its transfer across fluvial systems. Increase in concentrations and fluxes is general: at the finest scale, most major biogeochemical cycles are accelerated (erosion, N2 fixation, metal transfers). However, at the whole basin level, fluvial filters, either natural or anthropogenic, may greatly limit or even reverse these accelerations.
Most natural filters are either removed or greatly reduced in their functionality by human pressures (Fig. 2). The soil–vegetation filter has been modified since the beginning of forest cutting and crop agriculture. Wetlands are drained for agriculture and other land uses, floodplains are more and more isolated from the river channels by dykes and levees, estuaries are channelized for navigation and/or cultivated.

Fluvial system functioning in the Anthropocene. Net river fluxes to the coast depend on the balance between new sources of material (A,B,E,G,H,I,J), new filters as reservoirs (F6) and irrigated fields (F7) and reduction of natural filters as wetlands (F2), floodplains (F4) and estuaries (F5) by agriculture (AGRI), urbanization (URB), fluvial transport (TRANSP) and energy production. Irrigation (C) and interbasin water transfers (D) may greatly reduce river flow and all related fluxes. New sources include agrochemicals (A) (fertilizers, pesticides), infiltration and leaching of diffuse contaminants (B) brought by long-range atmospheric pollutants (LRAP) and by local atmospheric sources (J), irrigation returns (E), treated sewage (STP) from urban, mining and industrial sources (G), untreated wastes from the same sources (H), leaks from waste disposal sites (I). Downstream of reservoirs, river flows are regulated. Modeling of river systems and fluxes must integrate all socio-economic drivers and take into account the net material storage within the anthroposphere.
Fonctionnement des systèmes fluviaux à l'Anthropocène. Les apports nets de matière à la côte dépendent de l'équilibre entre les nouvelles sources de matière (A à J), les nouveaux filtres tels que les réservoirs (F6), les périmètres irrigués (F7), la réduction des filtres naturels (les zones humides (F2), les plaines d'inondation (F4) et les estuaires (F5)), sous la pression de l'agriculture (AGRI), l'urbanisation (URB), les transports fluviaux (TRANSP) et la production d'énergie. L'irrigation (C) et les transferts d'eau inter-bassins (D) peuvent diminuer fortement les apports d'eau et tous les autres flux. Les nouvelles sources comprennent les produits de l'agro-industrie (fertilisants, pesticides (A)), l'infiltration et le lessivage (B) des contaminants atmosphériques d'origine locale (J) ou lointaine (LRAP), le drainage des périmètres irrigués (E), les effluents traités (G) ou non traités (H) de sources minières, industrielles et urbaines, enfin les fuites du stockage de déchets (I). À l'aval des réservoirs, les écoulements sont régulés. La modélisation des systèmes fluviaux et de leurs flux doit intégrer les facteurs de contrôles socio-économiques et la rétention nette des matériaux dans l'anthropsphère.
In contrast, the lake filter function is so far not much altered at the global scale. Most world lakes, in number and in area, are located in the past-glaciated regions. In terms of lake volume, they are distributed between the tectonic lakes (e.g., Baikal, Tanganyika, Malawi, Victoria, Aral, and the Caspian Sea, the world's biggest lake) and the glacial lakes (North American Great Lakes from Great Bear to Ontario; Scandinavia, Taimir and Patagonia Lake districts) [40]. Most of these lakes basins are not yet exposed to important human pressures, excepted for lakes Ontario and Erie and for some localized pollution hot spots that may occur in these basins as for southern Baikal Lake [27]. The Aral Sea is very peculiar: in this endorheic basin, the environmental issues are linked to the massive water use for irrigation, which led to a total arheism for the Amu Darya and a very severe flow reduction for the Syr Darya [27,29].
In addition to these major but relatively slow changes, reservoir construction and expansion of modern irrigation works have been very rapid. Both have occurred mainly since the mid-20th century. The total reservoir area is estimated to be around and the total irrigated area to [62], compared to for the natural lakes of the world (excluding the Caspian Sea) [40]. Both reservoirs and irrigated areas should be explicitly considered in river flux modeling as they are very efficient filters for particulate matter and biogeochemical reactors (Fig. 2). The world's reservoirs are now counted in the hundreds of thousands [35] and their total sediment retention capacity is estimated to at least 30% of pre-impoundment fluxes, based on the major reservoirs only [78]. Their impact on nutrient retention, as total nitrogen, is also important: in North America, Asia and Africa, this efficiency of nitrogen transport within basins has decreased between contemporary and pre-industrial estimates [23] (Table 3).
Most small and many medium rivers are already very much affected by reservoirs, particularly in the Northern Hemisphere [17], but some of the largest world rivers are not yet affected. The impacts of reservoirs on river functions and on the Earth system are multiple and can be described in terms of flow regulation, longitudinal disconnection for the river biota, sediment imbalance, pollutant settling, nutrients processing and eutrophication [18,30,44,74,78]. Some of these impacts extend thousands of kilometers downstream (distal impacts or teleconnections) in the coastal zone as coastal sediment starving and erosion, coastal dystrophy [52], sediment blanketing of coral reefs, etc.
It is yet difficult to assess the exact influence of reservoirs on river fluxes, which depends on each type of material. On the basis of existing global scale studies [17,78], it can be postulated that about half of the stream water generated in basins headwaters is passing through one or several reservoirs filters further downstream in the river network.
Irrigation, which is sometimes associated with water diversion from one basin to another, is another new and essential filter at the continental surface. Due to water evaporation and other transference losses, targeted transpiration, nutrient uptake by crops and particulates settling in irrigated fields, fluvial systems affected by irrigation have much lower transport potential than under natural conditions. Some of them are not any more connected to their natural end points, thus also mimicking a response to a drier climate. Such ‘neo-arheism’ [44] is now developing fast: the Colorado River and the Amu Darya are some of the most typical examples with the Nile and Syr Darya. In these systems, the natural flow has been reduced by more than 80%. A 50% to 80% reduction of flow is also observed in many dry and semiarid countries as the south Mediterranean rivers from Spain to Turkey and Greece, the Rio Grande, Indus, Orange, Murray Darling, Huang He, the Black and Caspian Seas tributaries from the Dnepr to the Volga [27,73,74]. The exact development of neo-arheism remains to be established, however this task will be difficult for two reasons: (i) the gauging operations at the river mouth (i.e. downstream of many irrigated deltas) are not usually performed; (ii) when they exist, these data are not transmitted at global or regional data centers, as the GRDC [71,72]. Altogether the global area affected by significant river flow reductions () due to irrigation is probably exceeding .
A first qualitative estimate of the major Anthropocene changes of fluvial waterscape is presented in Table 2C. As for the natural variability of fluvial filters since the LGM, these changes are also very variable across major basins. So far, the Congo, Amazon and Orinoco Rivers are among the least impacted, while other systems such as the Colorado, Volga, Nile and the Bay James basin are completely engineered and controlled by humans. The exact position and nature of these new filters and the alteration of natural ones should now be determined at the basin scale.
7 Hot spots of riverine fluxes in the Anthropocene
Hot spots of riverine fluxes are a common feature of impacted basins. One single mine, factory, or a megacity without appropriate sewage treatment can release enormous fluxes equal to those found in the rest of the river basin or to a whole region [41].
On the Rhine Basin, one single potash mine in Alsace has discharged for several decades 10 000 to 15 000 metric tons of NaCl daily into the river corresponding to natural fluxes generated on basins 20 to 50 times bigger. Between 1978 and 1983, one single fertilizer plant in northern France discharged phosphoric acid residues into the small Espierre River that increased its natural P level by four orders of magnitude, thus being one of the main P supplier to the North Sea at that period. Many other pollution hot spots have also been documented, many of them are linked to mining and smelting and can be located in remote areas [27], otherwise pristine (Canada, Chile, Siberia, Papua New Guinea, etc.). Megacity wastes also correspond to hotspots, even when appropriately collected and created: the Paris megacity (, 107 people) is generating after sewage treatment a nitrate and phosphate flux equivalent to about one million square kilometers of forested western European basin prior to agriculture and urbanization [41].
At the regional scale, hot spots of anthropogenic fluxes have also been described, particularly for nutrients. All models of contemporary N and P river fluxes show a highly variable global picture, with yields ranging over two orders of magnitude, depending on natural conditions and on human pressures. When aggregating these fluxes at the continental scale, this heterogeneity is still visible. The total nitrogen fluxes to the oceans have increased 4.15 times faster in Western Europe (×6 increase) than for South America, where they have remained very close to their background levels (×1.2 increase) (Table 3). India, China, western Europe and western and central USA are the major global hot spots of nitrogen and phosphorous in contemporary conditions [73].
The collection and identification of hot spots of fluvial contamination remain to be realized at the regional to global scale.
8 Contrasted trajectories of riverine fluxes in the Anthropocene
Net global river fluxes to ocean have been greatly modified by human pressures, particularly over the last hundred years [73]. Yet the direction of these evolutions may be uncertain since three major changes must be considered together: (i) human activities have greatly accelerated the biogeochemical cycles and the global transfer of materials; (ii) fluvial system filters have been greatly modified, particularly through the creation of reservoirs; (iii) river water discharge to oceans is already controlled and reduced by water engineering and irrigation. Therefore, each type of river-borne material should be considered separately and many contrasting patterns [42] of evolution from the natural state can be defined, depending on the production/retention balance:
- – constant fluxes for few major ions (Ca2+, Mg2+, ) and particulate metals (Al, Fe, Ti, Na, K …) that are barely affected by anthropogenic activities, if river flow remains constant;
- – regular and moderate flux increase for some ions (Na+, K+, Cl−, ) in most rivers; increases are one order of magnitude faster in basins affected by mining;
- – fast increase for nutrients in some rivers since the 1950's, as nitrate and phosphate; while in other rivers bell-shaped trends are observed when pollution reduction has been successful (e.g., , and biological and chemical oxygen demands in the Rhine);
- – slow decrease of dissolved silica for biological uptake by river, lake and reservoir diatoms observed in the Mississippi River [52], western and northern Europe [11];
- – sudden occurrence of xenobiotic substances and general increase of metals in highly industrialized and/or mined river basins;
- – stepwise decrease of total suspended solids and their associated organic carbon, nutrients and contaminants due to reservoir storage after damming [37];
- – fast increase of total suspended solids after deforestation, particularly in mountainous wet tropics;
- – general decrease of all riverine fluxes resulting from irrigation and/or water diversion.
The global stability of some riverine fluxes may sometimes mask the fast evolutions occurring at regional to local scales. The particulate matter discharge to the ocean has been considered on a set of circa 150 world rivers, with a long record of sediment flux survey by Walling and Fang [83]. They found that most rivers do not show evidence of sediment flux increase, many of them being very large ones as the Mississippi and the Siberian rivers. On the other side, there is local evidence of a general increase of soil erosion and river particulate transport by factors ranging from 10 to 100 due to land use change. One typical example is the Huang He basin, observed over a long period through delta sedimentation records: the average sediment flux to ocean went from circa , one thousand years ago, to , in 1950–80, then down again to due to soil conservation measures [55,84]. In Taiwan, recent deforestation triggered an acceleration of erosion by a factor 10 and more [26].
Trends in sediment flux at the global scale are therefore very difficult to document [82]: (i) reservoir retention of sediments in fluvial systems is occurring at all spatial scales from hill-slope impoundments to the largest dam that may intercept upstream basins area of millions of square kilometers (e.g., Lake Nasser); (ii) the response of fluvial systems to anthropogenic erosion is best evidenced in the smallest basins (), while at the global scale, there is yet no sufficient data bank on these (notwithstanding the remarkable data set assembled by Milliman and Syvitski [48], who combined rivers of various drainage area, with and without human pressures). From their analysis, it is obvious that the most sensitive areas for sediment-flux increase to the coastal zone are the small and medium islands or coastal strips exposed to recent land use change and characterized by rugged relief. Some of these areas are also associated with the tropical regions where coral reefs are now exposed to sediment blanketing.
The global fluxes of some major ions (Na+, Cl−, K+, ), total nitrogen and nitrogen species, total phosphorous have already increased by factors 2 to 4 with regard to their pristine values (see a complete review in [73]).
The first estimates of global river fluxes were not spatialized. Most global nutrients and carbon budgets have then been spatialized according to oceans basins on the basis of major rivers inputs [8,9,48,60,61]. A new generation of budgets has used multiregression approach spatialized at 2° to 0.5° resolution [1,31,32]. Yet the routing of fluxes generated within the fluvial system could not be made. A next generation of model is now integrating the routing and takes into account some transfer functions for the major types of fluvial filters (soils, lakes, reservoirs, in-river processing …) [23]. Some models also explicitly combine all major processes throughout the river continuum on a seasonal basis, they were already tested on small () to large basins (e.g., Seine and Danube) [6,21]. In these models, biogeochemical transfer functions (e.g., bacterial degradation of dissolved organic carbon, denitrification in riparian wetlands, silica uptake by diatoms) are taken into account, sometimes through a stream-order analysis.
A very detailed description of fluvial functioning and riverine fluxes at a fine resolution is now possible, provided that processes, pressures and filters are well documented. The resulting river fluxes will have to be re-aggregated into regional entities, homogeneous for both the continental area and the receiving regional seas and marine basins. Such segmentation of the global coastal zone will facilitate the regionalization of ocean models.
9 Past and future trajectories of river fluxes
The functioning of fluvial systems can be directly determined on a relatively short period of actual river survey (1970–2000 for most river basins), a time during which human pressures have greatly evolved at the global scale, generally with increased impacts and related river degradation, sometimes with river recovery [42,44]. This documented period is used to establish and validate river flux models. The Earth system's study requires both the reconstruction of past river fluxes at different time scales (Holocene, Quaternary, geological times) and their projection into the near future, particularly the next hundred years [65,73].
The reconstruction of river fluxes in the recent past ( years) can be made by using historical data on pressures, land use, water use – as for the nitrogen budget in the Middle-Age Seine River [7] or using paleorecords in rivers and lakes sediments, or a combination of both as for the sediment fluxes in the Rhine, the Huang He, the subalpine Annecy Lake basins [50,82]. Longer Holocene variations of river fluxes will have to combine paleorunoff and paleodrainage reconstruction [2,22,73] with the current knowledge of the factors controlling river fluxes [1,31,32]. Use of geochemical tracers, like the Ge/Si ratio in aquatic biota for the past silica flux variation, is promising [20].
The projection in the next 50 years of both Climate Change and direct human pressures impacts of river fluxes models (Fig. 3) will be a different task. Present river fluxes should combine well-known global climate scenarios, and hypothetical, local basin-development scenarios [53,56,57,68,70,77]. These socio-economic scenarios take into account future watershed management [65,73,77] and land use strategies, waterscape changes (e.g., reservoirs, irrigation, diversion, draining, dykes) [74] as well as wastewater treatment and reduction of pollutant sources [47] (scenarios A1 to B3, Fig. 3). Few global scenarios have already been tested for river fluxes as the impact of a dietary change of the western world, from animal to vegetal proteins, on the global nitrate fluxes [61].

Trajectories of riverine fluxes during the Holocene and Anthropocene (accelerated timescale). Holocene variations, controlled by climate and sea-level rise, can be determined from paleorecords and extrapolation of models validated on present-day situations. For many fluxes, the contemporary state is already well outside the natural range since the Late Glacial Maximum, thus defining the Anthropocene era [12]. Anthropogenic emissions generally exceed fluvial system retention (e.g., nitrogen, phosphorous) (observed trend A). Reverse Anthropocene trends (B) can be observed, as for dissolved silica or suspended material in some basins. Scenarios of future fluxes should combine GCM scenarios for local river runoff and local socio-economic scenarios specific to each basin (water use, land use, wastes management …). Several trends are possible. (i) Lack of appropriate human responses to environmental changes (A1): the global evolution of fluxes is likely to continue in proportion of human pressures. (ii) Partial responses (A2, B2) with stabilization of fluvial systems, however at levels outside the Holocene range (e.g., for N, P, contaminants), therefore leading to some Earth system's changes. (iii) Global restoration of riverine fluxes to the Holocene range (A3, B3), an unlikely scenario considering the irreversible changes as land use and reservoir construction.
Trajectoires des flux fluviaux pendant l'Holocène et à l'Anthropocène. Les variations holocènes, contrôlées par le climat et la position du niveau marin, peuvent être reconstruites grâce aux archives environnementales et à l'extrapolation des modèles validés sur l'époque présente. Beaucoup de flux sortent déjà de la variation naturelle observée depuis le dernier maximum glaciaire, définissant ainsi l'ère Anthropocène [12]. Les émissions anthropiques excèdent en général les capacités de rétention du système fluvial (par exemple, azote, phosphore) (tendance A). Mais des tendances inverses (B) peuvent être observées (silice dissoute et, parfois, matières en suspension) : d'une part, les scénarios climatiques à l'échelle globale et la réponse hydrique locale ; d'autre part, les scénarios de développement socio-économique des bassins fluviaux (usages de l'eau et du sol, gestion des déchets, etc.). Plusieurs évolutions sont possibles : (i) une absence de réponse appropriée aux changements environnementaux (A1) – l'évolution des flux suit alors les pressions anthropiques – ; (ii) des réponses partielles (A2, B2) avec une stabilisation des systèmes fluviaux s'établissant à l'extérieur des variations holocènes (par exemple, azote, phosphore, contaminants), conduisant ainsi à un changement du système terrestre ; (iii) un rétablissement des flux à l'intérieur des variations holocènes (A3, B3), un scénario jusqu'ici improbable, en raison des changements irréversibles comme l'usage du sol et la construction de réservoirs.
River-basin scenarios are generally targeting the management of one issue (e.g., salinization, acidification, eutrophication, sediment balance). They should possibly also be able to combine these multiple issues occurring within one basin. In addition to the river-basin issues, some scenarios are now considering impacts of riverine-flux changes on the coastal zone at the local to regional level: e.g., coastal eutrophication, sediment starving of deltas or blanketing or coral reefs, persistent pollutant contamination [5,52,57,70]. Scenarios for the Earth system's future should also take into account the modified water, nutrients and toxicants balances in regional seas and oceans, regional climate change, and regional and global biodiversity [53,65]. Scenarios A3 and B3, which correspond to a global restoration of riverine fluxes back to the Holocene range, are unlikely, when considering pandemic anthropogenic activities, as land use and water resources management, for a large and still expanding human population.
10 Conclusion
Fluvial systems and their associated land–oceans fluxes are highly dynamic. The extrapolation of contemporary figures to the recent geological past or to the next several decades should be made very cautiously and take into account the following findings and questions.
- (i) Holocene variability. Over the last 18 000 years, fluvial systems have been essentially constrained by climate variability and sea-level rise. The land–ocean connection through rivers has been highly variable: the loss of river basin area due to the last glaciation has occurred together with an additional exposure of continental platforms to riverine transport. However, the location and nature of these continental areas are very different and it is therefore unlikely that these two phenomena are actually compensating each other in terms of global fluxes. Moreover, some now-endorheic regions of the continents were connected to the world ocean at some point since the LGM. Yet, the exact timing and space distribution of this connectivity remain to be determined at the global scale.
- (ii) Hot spots. More than 80% of river fluxes to oceans and to internal regions have been generated in less than 30% of the continents in natural conditions. Human impacts are increasing this contrast since major new sources of material are very concentrated in intensive agriculture areas, mining and industrial districts, megacities. Local to regional yields (i.e. expressed in ) may exceed global averages by one order of magnitude and more. Some of these river flux hot spots have been identified for both natural and Anthropocene conditions, yet many of them are not yet spatialized at a proper resolution.
- (iii) Natural fluvial filters. Fluvial systems fluxes are regulated by a set of natural filters that control the river material retention over various timescales. Their importance on river fluxes depends on their specificity and their relative positions within the system. The distribution of soils filters, headwater wetlands, slopes and piedmonts, inundated floodplain and estuaries may greatly differ from one system to another. The exact account of fluvial filters and their impact have to be quantified, particularly for hot-spot basins.
- (iv) Anthropocene evolution of fluvial filters. Over the last 100 years, fluvial systems have been largely impacted and modified by human activities and anthropogenic controls, which are now equaling the natural ones, thus defining the Anthropocene [12,42,44,78]. Many natural filters have been reduced, destroyed or disconnected, while new artificial filters were created, particularly the construction of reservoirs and the establishment of irrigation fields. The area directly affected by these changes is of the same order of magnitude than the area of natural changes observed over the last 18 000 years. Yet it is unlikely that the Earth system is already at equilibrium after this very sudden change, most of the impacts of global river changes being probably ahead of us, particularly for the coastal zone [65] and for the biodiversity [30].
- (v) Riverine fluxes trajectories. Many global river fluxes have already increased by a factor of 2, 3 or more with regards to their pristine values as for some major ions (Na+, K+, Cl−, ) as well as for nitrates, total nitrogen, and phosphate. Others are probably decreasing significantly, as for dissolved silica. The actual trend of river particulate fluxes is not easy to determine, precisely since it depends on the balance between accelerated soil erosion and accelerated reservoir retention. The direction and amplitude of these changes may greatly depend on specific river-basin conditions. Evolution rates, one order of magnitude faster, can be found as well as reverse trends. Because human impacts are adding a new complexity and spatial heterogeneity to natural processes, it is therefore essential to determine the present-day river budgets with the finest degree of spatial resolution and to begin considering explicitly in-basin processes.
Acknowledgments
This analysis has benefited from our participation to the BAHC and LOICZ IGPB projects and from financial support of the EU projects (Si-WEBS #HPRN-CT-2002-00218, Carbo Europe, #EVK2/2001/00307), and from University of New Hampshire from NASA SeaWIFS Project (NAG5-10260, NAG5-12451), Office of Naval Research (ONR) (N00014-01-1-0357), and NASA Interdisciplinary Science (IDS) (NAG5-10135). We are grateful to P. Houben (University of Frankfurt, Germany) for his helpful comments.