1 Introduction
So far, not a single satellite mission has been devoted to continental water, although a number of future missions, like CRYOSAT 2 or JASON-2, feature as a secondary objective to retrieve river stage and discharge or, as in the WATER project, have been primarily designed for continental waters. Over the last decade, the ongoing missions TOPEX/Poseidon (T/P hereafter), ERS 1 & 2, Jason and ENVISAT have contributed to the monitoring of continental water resources. The issues at stake are particularly important and will be reviewed in the first section of this paper. From the first report published by NASA on radar altimetry in 1969, to the current studies involving radar altimetry for river and wetland storage and various flow studies, the relentless efforts of the scientific community will also be reviewed in Section 2. Technical issues will be summarized in Section 3, dedicated to re-tracking processes, correction algorithms, multi-mission temporal series building, error and uncertainty estimation, and the geoid problem. The data thus processed can be still very useful for the hydrology community, and the applications of interest are discussed in the last section of this paper. This demonstrates the wide range of applications of satellite altimetry measurements today. This paper also presents the major advantages and drawbacks of hydrometric data used in hydrology today, either in situ or space borne.
2 The issues at stake
Freshwater supply in terms of quality as well as quantity will be a major problem in future decades. The world consumption of freshwater reached 54% in 1995 and is likely to equal the freshwater resource available by 2025 in North Africa and South Asia, when Asia will be using ten times more water than the rest of the world [59]. One billion people lack sufficient water for domestic consumption today, and it is estimated that in 30 years, 5.5 billion people will be living in areas with moderate to serious water shortages [45]. Water resource on the continental surface is limited and, additionally, its spatial and temporal distribution is inadequate. The volumes of water stored in the main reservoirs on Earth are as follows: 97.5% of the total amount of water is salt water making up oceans and only 2.5% is freshwater. Freshwater (68.7%) is mainly stored in glaciers and polar icecaps. Water resources most easily available for human consumption and the ecosystems are to be found in lakes and rivers, corresponding to only 0.27% of the freshwater and almost 0.007% of the total amount of water in the world [1]. Given our basic need for freshwater, the most important hydrologic observations that can be made in a basin are the temporal and spatial variations in water volumes in rivers, lakes, and wetlands. Unfortunately, our knowledge of the global dynamics of terrestrial surface waters is still very poor [56]. However, studies of the integrative, global nature of the hydrological cycle are essential to our understanding of natural climate variability and to predict climatic response to anthropogenic forcing [27]. Most international water management groups underline the need for core hydrological data. Monitoring of the continental water resource (temporal variability of river stages and river discharges) is provided via hydrologic networks. These networks are organized on a national basis. The challenges common to most regions include inadequate monitoring networks, gaps in records, a general decline in the number of stations, chronic under-funding, differences in processing and quality control, and differences in data policies [57].
The decreasing number of stage records available is shown in Fig. 1. The problem is twofold. The fall in stage records after ∼1980, reaching 0 for the 2000 period, is caused not only by a decreasing number of hydrologic gauges, but also by access time that may take several years in some cases. For example, WMO first identified those National Hydrological Networks in Africa that still maintain a hydrological data archive on paper. Replies to a questionnaire sent to Hydrological Advisers in 39 countries showed that 82 per cent use paper for archiving their data [58]. Thus, major issues in the poorer regions of the world include poor status or outright lack of monitoring networks and support infrastructure, and data quality. Spatial distribution of hydrological gauges around the world, as well as mean access time to data, is shown in Fig. 2, demonstrating the inadequacy of the monitoring capacity distribution in view of the distribution of the aforementioned freshwater needs.
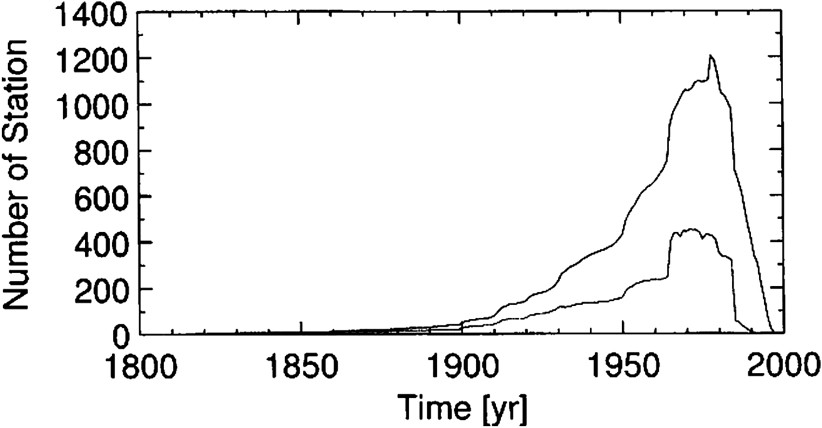
Decreasing number of water stage observations in the world from 1800 to 2000. It is worth noting that the dramatic decrease over the last years is due in part to the late data distribution.
Décroissance du nombre d'observations dans le monde entre 1800 et 2000. À noter que la chute brutale des dernières années comprend le délai de plusieurs années intervenant dans la publication des données.
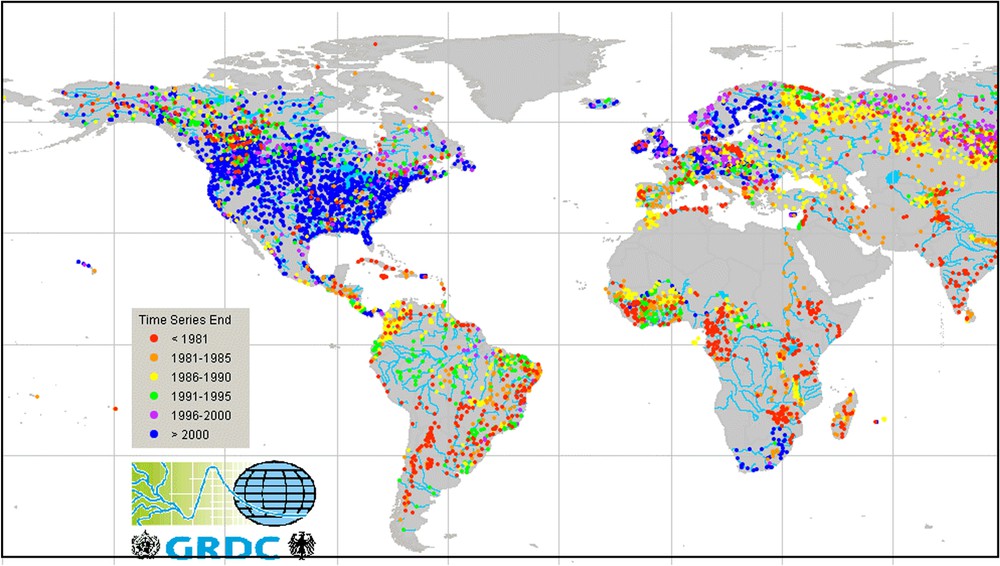
World distribution of the water monitoring capacity. http://grdc.bafg.de/servlet/is/1660/.
Répartition mondiale de disponibilité des données hydrologiques. http://grdc.bafg.de/servlet/is/1660/.
River and wetland water stages are integrating the basin response to climatic stress. The availability of raw data is key here. Presently, these measurements are much more reliable than the spatially averaged estimation of rain, evapotranspiration and infiltration. For example, Roads et al. [50] compared climate model outputs to ground-truth data over the continental United States. Using predictions from various climate models, they found that the runoff predictions are often in error by 50%, and even mismatches with observations as high as 100% were not uncommon. Also, Coe [16] found similar results for many of the world's large river basins. Thus, these models are now becoming limited as a result of the decline in observations of discharge and water storage [3]. As rivers, lakes and wetlands form the main freshwater resource, there is a need for a global, homogeneous, perennial monitoring system delivering fast-access data of continental water stages and, wherever possible, water volumes and river discharges.
3 State of the art
In 1969, NASA established the long-term objectives of the radar spatial altimetry, reporting the activities of the Williamstown group over solid earth and ocean physics [25]. In a report on SKYLAB altimeter results, Brown [12] analyzed the backscattering and typical waveforms of the Great Salt Lake of Utah. Miller [41] showed applications for the water stages of continental lakes from measurements collected by the GEOS-3 radar altimeter. Pioneer studies have used the data collected by Seasat in 1978 [11] and Geosat from 1986 to 1988 [43], demonstrating the feasibility of using data from ocean-designed missions for land waters. Mason et al. [36] conducted a prospective study of the ability of ERS1 data to measure the water stage variations in relation to climate changes.
On continental surfaces, radar altimetry left the prospective domain with the first studies dedicated to the Great Lakes in the United States, using SEASAT data [11], Geosat [43], and then T/P [4,42]. The African Great Lakes have also been the subject of various studies [5,7,15,38,39,46]. The first studies on great river basins began with Koblinsky et al. [26], who searched the specular GEOSAT waveforms to estimate the water levels on four sites on the Amazon. They estimated a 70-cm rms discrepancy between satellite and in-situ measurements, partly attributed to uncertainties in the in-situ record, but mostly to the uncertainty in the orbit determination. Orbit determination errors are decreasing with the new generations of satellite. From the aforementioned 50-cm error estimated for GEOSAT by Koblinsky et al. [26], the uncertainty in radial component of satellite orbits is now estimated to 15 cm for ERS-1 and 3 cm for T/P [34]. Now, data currently used to establish the time series of water stages over the world rivers originate from the T/P (10-day repeat period, since 1992), Jason-1 (10-day repeat period since 2002) and ENVISAT (following the ERS series started in 1991 with a 35-day repeat period) missions. Time series of river stages may cover a decade [5,6,19,35]. Satellite altimetry has also been used to determine the height profiles of rivers. Cudlip et al. [18] used SEASAT data to establish an Amazon water profile. They used 32 crossings of the river by the satellite tracks on an 18-day period in July 1978. These authors estimated the water-level accuracy to be between 10 and 20 cm. More recently, Birkett et al. [8] published time series of stage fluctuations over the Amazon Basin using T/P measurements between 1992 and 1999. It was concluded that accuracy may range from tens of centimetres to several metres (1.1 m of average rms). These authors found that the water-surface gradient of the mainstem varies both spatially and temporally, with values ranging from 1.5 cm km−1 downstream to 4.0 cm km−1 for more upstream reaches. Overall, they demonstrated that altimetric data from the T/P mission is successfully monitoring the transient flood waves of this continental-scale river basin. Recently, NASA has launched ICESat, a laser altimeter and data collected by the laser sensors (GLAS) seem highly promising [13,52], although no definite results have been published yet.
At present, various databases enable the retrieval of time series of water stage of the great basins of the world. The first database results from the processing of ERS and ENVISAT at the De Montfort University for ESA. Two types of product are currently available: the River Lake Hydrology product (RLH), designed for hydrologists with no prior knowledge of radar altimetry and organized in accordance with river/lake crossing points, each product corresponding to one crossing point; and the River Lake Altimetry (RLA) product, for radar altimetry experts. It is structured around orbit or by 35-day cycle and provides all crossing points for a predefined region [21]. The second database has been created at LEGOS, Toulouse, France, partly within the framework of the CASH project (Contribution of Spatial Altimetry to Hydrology) funded by the French Ministry of Research and Technology. It collects observations on rivers (∼200 sites) and ∼150 lakes in the world, mainly from T/P [30].
4 Technical aspects
Land-water investigators make use of dataset primarily intended and processed for other scientific purposes, namely to measure heights of the ocean surface (T/P, Jason, GFO, part of ERS 1 & 2 and ENVISAT) or ice caps (ERS 1 & 2, ENVISAT, ICESat). Using these data turns out to be difficult. Firstly, there is the width of the beam. As far as ocean-oriented missions are concerned, the beam is several kilometres wide, since surface average is needed over oceans to reduce as much as possible height measurement corruption due to wind waves. The drawback for hydrology studies is that echoes over rivers whose width is less than 1 km – that is, most of them – are noised by energy reverberated by riverbanks or islets. The second problem is the spread over time of the energy received at the satellite antenna. Over the rivers, the shape of the return waveform is much more different than over the ocean (Fig. 3). Smith [54] stated that the major difficulty in retrieving ranges over continental waters results from the variability in shape of the return waveforms when onboard trackers are designed for a typical ocean waveform. Regular ‘ocean-type’ trackers expect long tail shapes of energy distribution (view A in Fig. 3) when echoes bouncing off rivers are often specular [24] or a combination of specular echoes (view B in Fig. 3). Thus, in the best case, energy is received, but the range estimate is erroneous or not estimated (views C and D in Fig. 3); in the worst case, the altimeter loses tracking and subsequent echoes are lost. The antenna-reflector range is determined by fitting the waveform with a predefined analytical function (so-called waveform ‘tracking’). If the analytical function is not well suited to the waveform shape, tracking leads to wrong estimates of the height value (or even no estimate at all). It is worth noting that the radar altimetry data collected by the ongoing ENVISAT mission are nominally retracked with four algorithms [10,23]. In turn, retracking the radar waveforms collected by the ERS 1 & 2, T/P, Jason, and GFO missions requires that some retracking procedures be conducted. These cumbersome tasks are being carried out, particularly with De Montfort University in England, LEGOS in France, OSU in the USA, among others. The third issue is mispointing. Indeed, when the area at the nadir of the antenna is not reflecting much radar energy, the energy bounced by a water surface at the edge of the footprint can dominate the echo returned, although damped by the antenna pattern. The tracking procedure will then determine the range by fitting this slant return of energy. Because the range estimate assumes that the target is at the satellite nadir, this leads to an overestimated range, which is an underestimate of the height of the reflecting water surface. This is not uncommon in continental waters and has to be carefully taken into account, because these measurements can be erroneously attributed to some hypothetic wetland in the vicinity of the main reach of the river sampled. An example is given in [23]. The third geometrical effect impacting accuracy is the slope effect. Indeed, within a radar footprint a few kilometres wide, the river height can change significantly. This changes the waveform shape and affects range determination. Also, in braided reaches, branches may have different heights, which will spread the return time of energy and contribute to an erroneous range determination.
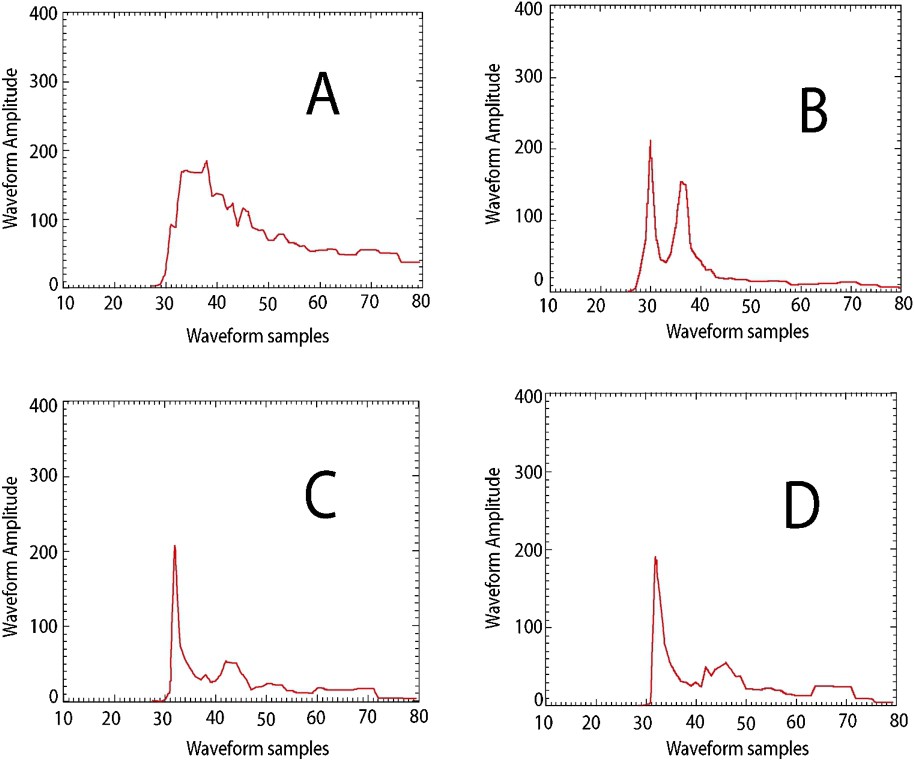
Samples of TOPEX waveforms over rivers in the Amazon Basin. A: ‘Ocean-like’ waveform processed by the onboard tracker. B: Multi-peak waveform rejected by the tracker. C: Specular waveform processed by the tracker. D: Specular waveform, similar to C, rejected by the tracker.
Exemples de formes d'onde Topex sur les rivières du Bassin amazonien. A : Forme d'onde de type océanique traitée par le tracker de bord. B : Forme d'onde à plusieurs pics, rejetée par le tracker de bord. C : Forme d'onde spéculaire traitée par le tracker. D : Forme d'onde spéculaire, quasi identique à C, mais rejetée par le tracker.
Lastly, the air density, the amount of water vapour and the content of free electrons in the ionosphere modify the travel time of radar waves throughout the atmosphere. The electronic contents in the ionosphere and air pressure are given by independent datasets. However, the amount of water vapour is estimated using microwave radiometers embarked onboard together with the radar altimeters. The current microwaves radiometers fail to estimate the atmospheric content of water vapour over continents because the signature of the atmospheric water vapour is mixed with that of ground wetness. Thus, this effect, ranging from a few centimetres to tens of centimetres, cannot be accurately corrected for over land waters and corrections can only be estimated from large-scale global datasets such as ECMWF.
The time step of the altimetric series is given by the orbit repeat period. For the current radar altimetric missions, this period ranges from 10 days for T/P and Jason to 35 days for ERS-2 and ENVISAT. GFO has an intermediate repeat period of 17 days. In terms of sampling rate, this is much lower than the time step of in-situ measurements usually collected once or twice a day. Although no studies has been conducted to evaluate the amount of information lost due to this under-sampling, clearly some applications of water level monitoring must be discarded when dealing with the altimetric time series of the water level. To improve this time step, multi-mission series can be constructed in some ‘fortunate’ places where satellite tracks intersect with a river reach, thus creating a joint ‘virtual station’. An example of such series is given in Fig. 4. These combined series raises the issue of the type of errors in data series. Indeed, Calmant and Seyler [13] have shown that the error budget of each mission includes biases that must be accounted for prior to combining the data from different missions. Several studies have dealt with the estimation of the error budget in the altimetric series of continental water stages. In particular, Birkett et al. [8] compared the altimetric series with in situ series. Discrepancies up to several metres have thus been reported. However, it should be pointed out that these comparisons suffer severe limitations. On the one hand, the satellite track usually fails to cross the river right over a gauging station. Comparing both series means that the water stage varies identically in both places, but this is often not true. Indeed, water stage variations along a reach are affected by changes in reach width. Also, flow routing should be performed to account for the delay from one place to the other when the distance between both locations is significant with respect to flow velocity and sampling rate. Typically, the delay is one day – e.g., the sampling rate of gauging stations – for a flow to cover 85 km with a 1 m s−1 velocity. Given that water stages tend to vary by several centimetres or decimetres within a day, the error induced when the delay is not taken into account can contribute significantly to the discrepancy between both series. On the other hand, when the gauge is not levelled, scatter between altimetric and in-situ series is computed with reduced – zero mean – series, and the resulting estimate of the discrepancy does not take into account possible biases, such as the tracker-dependent bias as long as these are not tabulated. Alternatively, the accuracy of altimetric measurements can be assessed by means of a comparison with GPS ground truth height measurements right under the satellite track. Although this technique is widely used to assess the accuracy of altimetric data over oceanic surfaces ([10], in particular), this kind of fieldwork has seldom been conducted over rivers and results published, as in Frappart et al. [23], are too limited to allow definite conclusions to be drawn as to the determination of the error budget in altimetric series using this approach. Although intrinsic system errors of radar measurements are the same over land waters and oceans, e.g., centimetre accuracy, the overall uncertainty of altimetric measurements over continental waters is now in the order of a couple of decimetres [8,13,14,23].
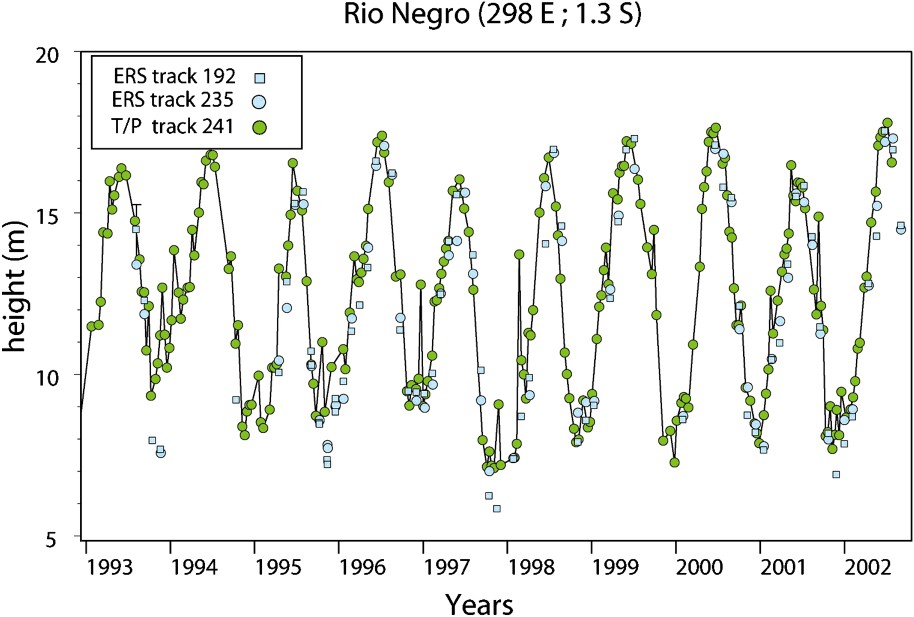
Multi-mission (ERS-1 & 2 and T/P) series of water stage over the Rio Negro: combining datasets from different satellite missions enables us to diminish the average time step in the series.
Série temporelle composite ERS-1 & 2 et T/P de niveau d'eau sur le rio Negro : la combinaison de jeux de données issues de différentes missions satellitaires permet de réduire le pas d'échantillonnage temporel moyen dans la série.
Many hydrology laws used to model flow routing include the river slope with respect to the geoid to account for the actual gravity contribution to the flow velocity. The quality of geoid determination may vary greatly from one place to another. In some countries, such as in Europe or Northern America, the geoid undulations at the kilometre scale have been determined, but in most other countries, including those with the largest river run, gravity measurements are sparse and only global scale geoid solutions can be used. These global models such as EGM96 [31], GCM01C or GGM02C [55], for the most recent ones, merge in-situ gravity measurements and space borne ones. Space borne determinations of the geoid were mostly derived from orbit perturbations. The launch of the GRACE satellite mission in 2002 [55], primarily dedicated to the measurement of the geoid and of its temporal variations, led to a much greater accuracy for the determination of geoid wavelengths up to . Shorter wavelengths are only determined by local measurements, and thus, remain almost undetermined over the largest basins. As a result, river slopes with respect to the geoid are only known at a large scale and flow routing at a small scale designed to evaluate river discharges in these basins is still tentative. This problem is illustrated in Fig. 5, where the ICEsat ellipsoidal height measurements [60] over the Tapajos River are converted to altitude with respect to the GGM01C and GGM02C solutions of global geoid. GGM01C is a purely GRACE solution when GGM02C is a combination of long-wavelength geoid from the GRACE mission and short-wavelength geoid from the EGM96 solution. Clearly, the river slope differs significantly in both instances, with dramatic consequences for the hydrologic regime, since the minimum altitude is not at the river mouth for the profile estimated with respect to GGM02C. It is worth noting that the solution with respect to GGM02C best explains the peculiar hydrology of this part of the river (J.-L. Guyot, personal communication).
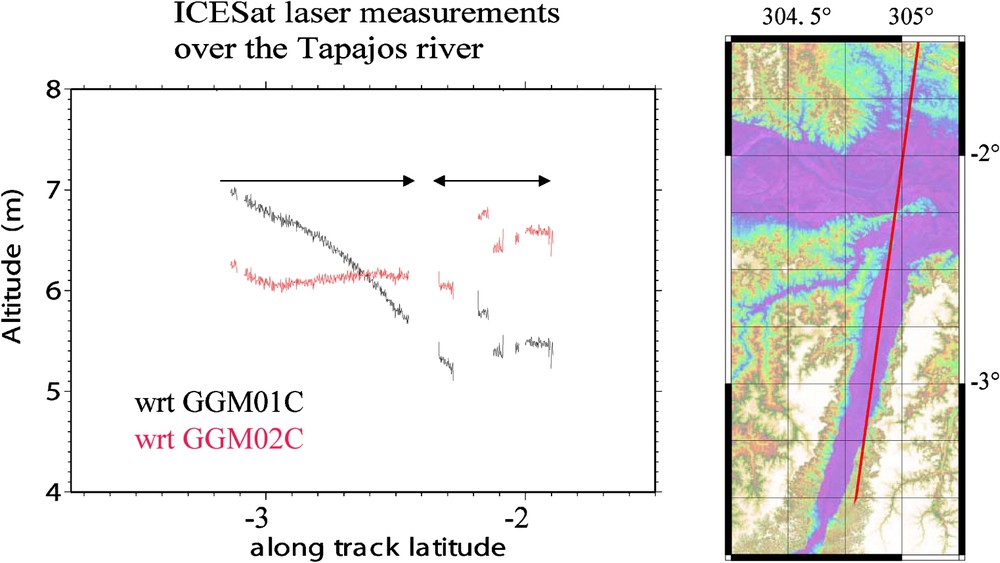
Altitudes of surface water along the Tapajos River. Height measurements collected by the ICESat satellite are referred to two GRACE solutions: GGM01C and GGM02C. The slope is very different according to the geoid model, with strong implications in flow modelling.
Altitudes de la surface de l'eau le long du rio Tapajos. Les mesures de hauteur ellipsoïdales collectées par le satellite ICESat sont rapportées à deux solutions de géoïde issues de la mission GRACE : GGM01C et GGM02C. La pente de la ligne d'eau est très différente selon le modèle de géoïde, avec des implications importantes sur la modélisation du flux.
5 Applications
A range of applications derived from the altimetric measurements of continental water level has been performed or is currently underway. A brief review of these applications is given hereafter.
5.1 Levelling of hydrological network gauge stations
A number of great river basins are located in remote geographical areas. These basins include hydrological gauges, where topographic levelling has not been carried out or, in some cases, levelling uncertainty is too high due to the difficulties in conducting the conventional processes of terrestrial – spirit – levelling. By way of example, for the Amazon Basin, most hydrological gauges from the ANA network (Brazilian National Agency for Water) are located outside the topographic levelling routes of IBGE (Brazilian Institute for Geography and Statistics). Additionally, gauge stations within the hydrographical basin of the Amazon but in the neighbouring countries of Brazil are all unlevelled, apart from Iquitos station in Peru, which has an altitude measurement for the hydrological 0-gauge. This is a major drawback for hydrodynamic modelling of the basin, since this kind of models requires that the hydrographical parameters of the river, such as bed slope, be entered into the model by means of a common altitudinal reference. Kosuth and Cazenave [28] conducted a preliminary study of gauge levelling from T/P time series of water stage in the Amazon Basin. Cauhopé [14] levelled gauges in the Curuai Varzea and the adjacent Amazon reach using ENVISAT time series. From the Tapajos River, Calmant and Seyler [13] showed that ICESat measurements are particularly well-suited for this gauge levelling. In particular, altimetric measurements are an efficient tool for gauge levelling. However, levelling of the worldwide gauge networks has still to be conducted.
5.2 Estimation of discharge from stage altimetric measurements
Several works have examined the ability of spatial data to retrieve river discharge. Recently, Bjerklie [9] estimated in-bank river discharge on the basis of the hydraulic relationships constrained with remotely-sensed width information and channel slope obtained from topographic maps. Coe and Birkett [17] estimated the mean monthly river discharge of the Chari River at N'Djamena, Chad. They used T/P surface water stages upstream from the gauging station calibrated with the ground-based gauge height and discharge data using simple empirical regression techniques. Kouraev et al. [29] estimated discharge for the Ob River (Siberia) along two T/P tracks crosscutting the river in the vicinity of the Salekhard gauging station. T/P measurements were found to provide reliable water level (H) time series that can then be used to estimate water discharge (Q) from the rating curve between H and Q at Salekhard, located 65–70 km away from the T/P tracks. These studies suggest that remotely-sensed river hydraulic data could be used to directly estimate discharge at a specific location, if ground-based discharge measurements are used to develop discharge ratings in conjunction with the remotely observed variable(s). As pointed out by Bjerklie et al. [9], discharge ratings developed from ground-based flow measurements and remotely sensed hydraulic information are site specific. Leon et al. [32] developed a model based on diffusion–cum–dynamic wave propagation assumption, using in-situ discharges and radar altimetry data to estimate rating curves at the satellite track crossings in the Negro River Basin, Amazon. The calibration phase led to differences of less than 4% between measured and estimated outflows and validation has yielded less than 10% errors.
By estimating discharges from altimetric water stages and in-situ discharge, denser stage-discharge observations within a basin can be obtained. For example, out of the 571 gauges listed by ANA, 46 are located in the Negro River sub-basin and 25 have complete records covering the last 20 years. Along the T/P tracks, water level time series were built for 88 T/P crossings with river and floodplains [22]. Based on altimetric heights, 3.5 times more measurement points are available in the Negro River Basin. These points are evenly distributed within the basin, allowing for a regionalization of water fluxes.
Denser stage and discharge estimations entail many implications. For example, it is essential for water management, extreme flow prediction, and hydrological modelling. Rain gauges within remote watersheds are often poorly distributed, although the spatially averaged estimation of rain is an important parameter of Global Climate Models. As previously pointed out, a better distribution of the integrated response of the basin to the incoming rain allows a better validation of these models. Better-distributed fluxes could also be used to constrain models of weathering processes and carbon flux estimations.
5.3 Estimation of the spatial and temporal variations of water storage: rivers and wetlands
As pointed out by Alsdorf et al. [3], “for the past 100 years, our understanding of the hydraulic characteristics and hydrologic mass-balances of surface water runoff have largely been derived from discharge measurements at inchannel gauging stations. Measurement of inchannel discharge unfortunately does not provide with the information necessary for understanding flow and storage in off-river-channel environments, such as wetlands, floodplains, and anabranches (e.g., braided channels); these environments are increasingly recognized for their important roles in biogeochemical cycling of waterborne constituents”. Using radar interferometry, Alsdorf et al. [2] estimated water height changes in an Amazon lake to be about , and a volume change of during the 44 days between the two JERS images used. These authors reviewed a T/P crossing of the lake and estimated the change in water stage to be of . The T/P nadir measurements might appear less accurate than interferometry, but so far, only these measurements offer 10-day periodic estimates over more than a decade. In an extensive study of T/P measurements over the Amazon Basin, Birkett et al. [8] have successfully distinguished river from floodplain in a number of cases. A small phase offset of a few days in stage variations between river and nearby floodplain had occasionally been observed. Frappart et al. [22] determined spatio-temporal variations of water volume over the main stream and floodplain located in the Negro River Basin, using area variation estimates for a seasonal cycle captured by the Synthetic Aperture Radar (SAR) onboard the Japanese Earth Resources Satellite (JERS-1), and changes in water level from the T/P altimetry, combined with in-situ hydrographical stations. A volume variation of 331 km3 was estimated for the whole Negro sub-basin, enhancing the complex relationship between the volume potentially stored, the inundated area and the volume flow during the same period. Ramillien et al. [47,49] devised a robust inverse method for unravelling the hydrological contributions of the main water mass reservoirs to the time-variations of the gravity field from the monthly geoids made available by the CSR (University of Texas, Austin, USA) and GFZ (Potsdam, Germany). Clearly, both approaches are complementary and the method developed by Frappart et al. [22] to estimate seasonal variations of surface free-water volume could be combined with the time-series of continental water storage variations between April 2002 and June 2004 presented by Ramillien et al. [48] to analyze seasonal water mass transfers at a regional scale.
5.4 Water profiles and geodynamical implications
River free-surface slope is an important parameter in floodwave propagation models and sediment transport. For example, slope values of a few centimetres per kilometre were evaluated for the Amazon mainstem using barometric estimates of elevation performed at some gauging stations [37,44,51,53]. Guskowska et al. [24] and Cudlip et al. [18] used 15 out of the 32 crossings of the Amazon River by the SEASAT altimeter to provide an estimate of the elevation profile of the Amazon, whereas Mertes et al. [40] and Dunne et al. [20] used the SEASAT falling stage measurements to calculate 14 gradient values for the Amazon mainstem. Birkett et al. [8] have used T/P measurements along the mainstem of the Amazon to estimate the spatial and temporal variation of the gradient values. The slope of the riverbed is an important parameter for modelling river hydrodynamics. Recently, Leon et al. [33] have proposed a methodology based on the georeference of altimetric data to derive along stream profiles of riverbed height and slope. The height of the river bed at so-called virtual stations is determined as that height at which discharge vanishes in rating curves established by combining times series of water height by satellite altimetry with discharges predicted by routing the flow recorded at remote in-situ gauges.
6 Conclusion
The major drawback in the use of altimetric height for water stage monitoring is the temporal sampling rate. Clearly, the 10-day period of T/P and Jason and the 35-day period for ENVISAT cannot compete with observations made daily or twice a day in most of the in-situ gauges around the world. In some applications like flood events, the models require even more frequent data, of the order of the minute. Another limitation is measurement uncertainty, which has been seen to vary from a few centimetres to the metre in the worse cases. With respect to radar altimetry, accuracy can, to a certain extent, be improved by adapting the processing of echo waveforms to the continental case. This uncertainty is due to the ground point target size, ranging from kilometres for T/P to a few hundred meters for ENVISAT and 70 m for ICESat, with a decreasing uncertainty, as the combination of water and vegetation or the merging of different water bodies in a single footprint becomes less likely. When it comes to identifying and separating peaks of energy reflected by small water bodies, the sensor and echo processing capability becomes a major issue. A resolution of approximately 100 m can be expected in future missions.
However, altimetric data offer many advantages. Among them is the ability of tracking either inchannel fluxes or flow and storage in off-river-channel environments, such as wetlands, floodplains, and anabranches. These are critical for surface water balance. The development of methods to estimate the river discharge using remotely-sensed data would provide the means to increase the streamflow measurement network globally. Typically, in-situ data collection and management activities are undertaken at the national level, where there is a need for regionally-coordinated systems and actions. Worldwide coverage and near-real time availability of measurement is definitely another major benefit of satellite altimetry with respect to in-situ measurements.
The prospects offered by future missions like CRYOSAT, carrying a SAR interferometric altimeter onboard, Altika, carrying a narrow footprint beam in Ka band or the WATER project, submitted to the European Space Agency (ESA) and especially dedicated to the monitoring of continental waters (WatER Homepage: www.geology.ohio-state.edu/water), will definitely enhance the ability of spatial data to be included in models and change the management and monitoring of water resources. Clearly, satellite altimetry is not likely to replace in-situ measurements in the near future, but a combination of both systems will certainly enhance our ability to monitor the cycle of surface water from the regional scale to the world at large.
Acknowledgements
The authors are grateful to P. Bates for reviewing the manuscript.