1 Introduction
Irradiation encompasses a large diversity of interaction modes of accelerated particles with solid or gaseous matter. Depending on the energy of the accelerated particles, irradiation can lead to structural damage (e.g., [80]), or to the production of new isotopes via nuclear reactions (e.g., [19]). Here we will restrict ourselves to nuclear effects, i.e. to the nucleosynthesis production of new isotopes due to the interaction of matter with MeV cosmic rays, and more specifically on the nucleosynthesis of short-lived radioisotopes (SRs) in the early solar system. Other effects of irradiation are discussed in a variety of recent reviews [5,7].
Short-lived radioisotopes (SRs) are radioactive elements with half-lives of Ma. Their presence in the nascent solar system is inferred from excesses of their daughter isotopes in meteorites’ components, mostly in primitive components such as Calcium-Aluminium-rich Inclusions (CAIs) [64,77]. Some SRs (see Tableau 1) were present in the protoplanetary disk at abundances significantly higher than the expected average contribution of the interstellar medium [53]. These SRs therefore require a last minute origin (e.g., [75]). The origin of SRs is highly debated in the cosmochemistry community, as it has important consequences for the early solar system chronology and planetesimal heating (e.g., [28,74]). Elucidating the origin of SRs can also potentially constrain the astrophysical context of our solar system birth (e.g., [29,56]).
Initial values of radionuclides considered in the present paper
Tableau 1 Valeurs initiales des radionucléides considérés dans le présent article
R | T1/2 (Ma) | D | S | R/S | ref |
53 d | [8] | ||||
0.1 | [67] a | ||||
0.3 | [36,46] | ||||
0.74 | [79] | ||||
1.5 | [51] | ||||
1.5 | [54,70] | ||||
3.7 | [2] |
a The initial value of was calculated from the measured initial / [67], and assuming it endured an isotopic resetting similar to that of [27,31,52].
Generally speaking, SRs can be made either by non-thermal nucleosynthesis (nuclear reactions implying cosmic rays at MeV energies), or by thermal nucleosynthesis (nuclear reactions at keV energies in the interior of stars). Short-lived radionuclides once present in the solar protoplanetary disk therefore originated from late-type stars delivering freshly made nucleosynthetic products (e.g., [4]), or by in situ irradiation of solar system dust or gas (e.g., [31]). As far as the stellar model for the origin of short-lived radionuclides is concerned, supernovae are the most likely candidates, because Asymptotic Giant Branch (AGB) stars are unlikely to be found in the vicinity of star-forming regions [37].
Among short-lived radionuclides, some play a special role, such as and the radioactive beryllium isotopes ( and ). Because cannot be made by irradiation [44], it is often asserted that the past presence of in meteorites is definite evidence for the contamination of our nascent solar system by a supernova ejecta (e.g., [36]). This opinion is tied to the notion that the initial solar system / ratio was high (, Tableau 1). Be isotopes are destroyed in the interior of stars [61] and cannot have a stellar origin. The irradiation origin of is well established; however, its presence in the early solar system was reported only from one CAI [8]. Beryllium-10 was ubiquitous in the formation region of CV3 and CM2 CAIs [8,48,49,51,68]. The early solar system is attributed to irradiation by a majority of authors ([6,7] and references therein). Desch, Connolly and Srinivasan [10] challenged however the idea that the discovery of beryllium-10 was a ‘smoking gun’ for internal irradiation in the early solar system [26].
The goal of the present article is to establish the irradiation origin of (Section 2), to review the irradiation models leading to the production of other SRs together with beryllium isotopes (Section 3), to examine the (indirect) evidence for early solar system irradiation given by X-ray observations of young, solar-like stars (Section 4), and to discuss the initial abundance and origin of iron-60 (Section 5).
2 The irradiation origin of
The first evidence for beryllium-10 in the early solar system was brought by [51]. Since then, numerous authors confirmed that beryllium-10 was abundant in the protoplanetary disk, at least at the time and spatial location of CV3 and CM2 CAIs formation [51,68,48,49,8]. Beryllium-10 is produced vastly by any irradiation model [25,45,50], and since its discovery, its presence in the early solar system has been ascribed to the irradiation of nebular dust or gas by protosolar cosmic rays (e.g., [7]). Desch et al. [10] challenged that idea, and proposed that most of the beryllium-10 observed in CAIs was made via Galactic Cosmic Ray (GCR) trapping during the molecular cloud core stage which precedes the formation of a protostar. In the following, we examine this proposal at the light of molecular cloud cores observations.
The amount of trapped in a molecular cloud core depends mainly on the GCR flux, on the core surface density and on the core lifetime [10]. To reproduce the observed solar system abundance, Desch et al. [10] need a factor of 2 increase of the GCR flux. The GCR flux is directly linked to the star formation rate, and there is, at present, no experimental evidence for an increase of the star formation rate 4.5 Gyr ago [63]. To model the core surface density, they use ambipolar diffusion models developed by [9]. Ambipolar diffusion models are now known to poorly describe the evolution of a molecular cloud core (e.g., [38,41]). We note, en passant, that the core considered by Desch et al. weighs 45 M⊙, one order of magnitude larger than the cores which give birth to solar-like stars [10]. Finally, to trap enough , the molecular cloud core needs to trap for Myr [10]. This timescale far exceeds the observed timescales of molecular cloud cores which are typically of a few yr [55]. 1 Myr is probably a maximum value for the prestellar core lifetimes [39–41,55]. It is also worth noting that there is now a wealth of observations and theoretical considerations suggesting that molecular clouds do not leave longer than a few Myr (e.g., [1,13]). Cores which are a subentity of molecular clouds can hardly have a lifetime longer than the molecular clouds themselves. Given that the conditions needed to reproduce the abundance of in the early solar system strongly differ from those observed in observed molecular cloud cores, it casts some doubt on the trapping hypothesis.
Given the difficulty of trapping from the GCR in the molecular cloud core, and the facility to make it via irradiation, it is probable that most of the present in the early solar system originated via the irradiation of gas and dust by protosolar cosmic rays.
3 Co-production of other short-lived radionuclides by irradiation
3.1 General considerations
As demonstrated above, it is likely that most of the found in CAIs is made by in situ irradiation in the early solar system. Among other short-lived radionuclides, and are also very likely to originate from in situ irradiation for the reasons given below.
Beryllium-7 was discovered in the Allende CAI 3529-41 by [8]. Beryllium-7 is a SR with an extremely short half-life ( days). Such a short half-life prevents a SN injection in the solar system. Timescales separating the end of nucleosynthesis in SN and incorporation in CAIs are estimated to be of Myr [29,56]. After Myr, all the putatively made in a SN would have decayed. In addition, stars do not produce beryllium isotopes [61].
Evidence for was found recently in a diversity of Allende and Ningqiang CAIs [36,46]. Not only does the early solar system abundance of relative to that of far exceeds that of supernova ejecta [36,59], but it is also virtually impossible to mechanically inject into the protoplanetary disk via a supernova. Ouellette et al. [57] showed that injection of SRs by a SN occurs in the solid phase. The gas phase of the ejecta is deflected by the high density disk, implying that only the elements locked in solid dust grains can make it into the disk. It is, however, well known that chlorine resides in the gas phase in the interstellar medium [65], and that there is no known refractory mineral that can incorporate chlorine [47]. Combined with the fact that dust condensation is inefficient in supernova ejecta [14], it means that virtually no chlorine-36 atom made in a supernova can make its way into a nearby protoplanetary disk.
More generally, from the astrophysical point of view, Gounelle and Meibom [30] showed that it is extremely unlikely that a supernova exploded close enough of a newly formed planetary disk to inject the correct amount of SRs. This is mainly because massive stars evolution timescales are too long, compared to typical timescales of star formation in embedded clusters, for them to explode as supernovae within the lifetime of disks, which will be limited by UV ionization (from the massive stars) and planetary formation.
3.2 Irradiation models
Nucleosynthesis via irradiation of matter in the solar accretion disk by high energy particles ( MeV) has long been considered as a promising alternative path to stellar nucleosynthesis [19]. The discovery that was alive in the solar accretion disk [42] prompted studies examining its production via proton irradiation (e.g., [35,43]). These authors estimated that large fluences were needed to reproduce the observed abundance. In the absence of experimental evidence for elevated fluences, irradiation fell into relative disgrace until Lee et al. [44] revived it (see below), and X-ray observations of solar-like young stars provided a strong astrophysical evidence for irradiation [17].
The main parameters of any irradiation models are the proton flux, the irradiation duration, the abundance of cosmic-rays () relative to protons (), the target abundance, the nuclear cross section, and the energy spectrum of protons [7]. The proton energy spectrum is usually considered to satisfy a power law, , with varying index p. The most likely astrophysical context for irradiation synthesis of short-lived radionuclides is the solar accretion disk [7]. In such a context, the Sun magnetic activity has the possibility to accelerate particles according to two broad classes of events [60]. Gradual events emit soft X-rays and are electron- and -poor. More frequent impulsive events emit hard X-rays and are electron- and -rich. Impulsive events have steeper energy spectra than gradual flares.
A model examining the possibility of producing short-lived radionuclides by irradiation of solar system dust by proton and nuclei at asteroidal distances was examined by [25,49,50]. In this work, shielding of the whole solar accretion disk is ignored and accelerated solar particles are supposed to have free access to dust at AU. These authors limit themselves to examine gradual events having shallow proton energy spectra () and normalize their yields to . Marhas and Goswami [50] contend that irradiation can account for all , 10 to 20 % of and and none of the present in the early solar system.
The irradiation model [26,31,44] in the context of the x-wind theory for low-mass star formation (e.g., [66]) introduced four conceptual modifications compared to the previously discussed models. First, irradiation takes place close to the Sun, in a gas-poor region, enhancing considerably the proton flux [44] compared to models that locate irradiation at asteroidal distances, where the proton flux is dramatically reduced by an inverse square law and the interaction with all the gas contained in the solar accretion disk. Second, it has opened the channel for the production of short-lived radionuclides. Opening the channel makes it possible to produce at a level comparable to what is observed in meteorites [44]. Third, it provides a natural transport mechanism, the x-wind, from the regions close to the Sun where irradiation takes place until asteroidal distances where chondrites are believed to have agglomerated [66]. Fourth, it calculates absolute yields instead of relative yields scaled to a given short-lived radionuclides. This is because the proton and other accelerated particles fluxes are scaled to X-ray young, solar-like stars observations rather than normalized to a given short-lived radionuclide such as [44].
In the x-wind model, irradiation of protoCAIs takes place in the reconnection ring where magnetic lines tying the protostar and the accretion disks reconnect, providing a powerful mechanism for accelerating , and nuclei to energy up to a few tens of MeV [44]. After irradiation has taken place, protoCAIs are picked up by the x-wind and delivered to asteroidal distances. In the favorite model of [26], / , / and . The proton flux is scaled to the X-ray young, solar-like stars observations using an empirical relation found for the present Sun, ( MeV) [44]. The average erg s−1value of young, solar-like stars estimated by [44] and used by [26] is very close to that found in 2002 by a survey of 43 low-mass stars in the Orion Nebular Cluster [16]. The irradiation time depends on the protoCAIs size and varies from to yr. The steep energy spectra () with elevated abundance corresponds to impulsive events in the Sun [60]. Using these parameters, Gounelle et al. [26] calculated that the initial solar system abundance of , and could be reproduced within a factor of a few. In that model, was overproduced compared to the measured initial abundance (/ ), unless a core-mantle structure for protoCAIs was considered.
Using the same exact model as [26], Gounelle et al. [31] could reproduce the initial abundance of measured by [8]. The ability of the x-wind model to produce a relatively high amount of relative to arises from low irradiation times, that can compete with the fast decay of . They showed that if abundance was originally higher than the measured value, as expected from recent work on [27,31,52,79], its initial abundance could be reproduced as well (Fig. 1).
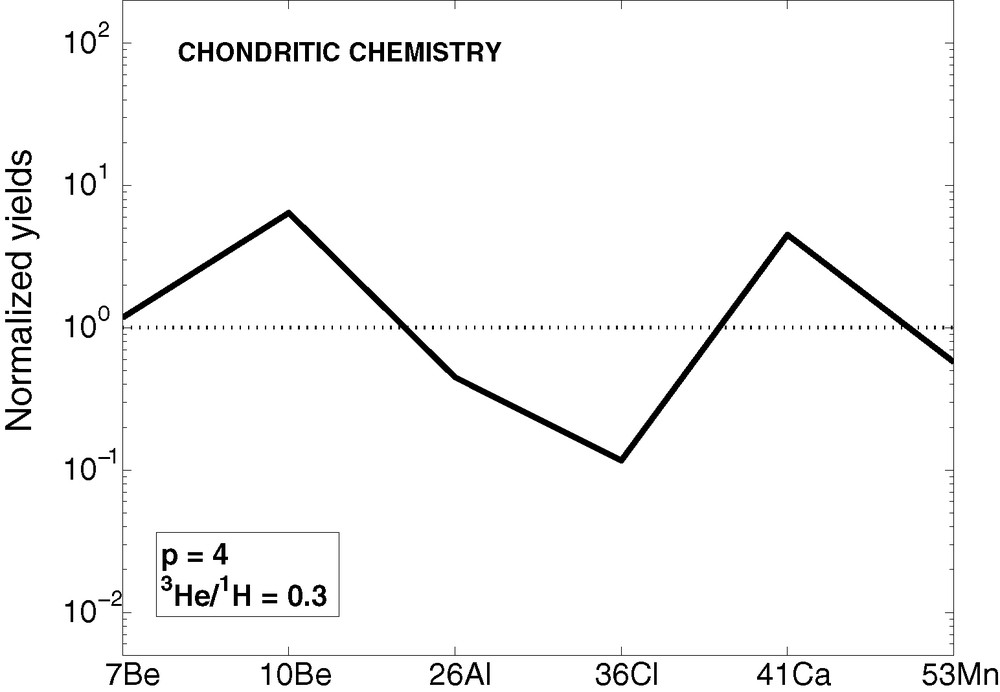
Results of the irradiation model of [31]. Yields of , , , , and for a chondritic chemistry and the spectral parameters and / . The yields are normalized to the values mentioned in Tableau 1. (Adapted from [31]).
Fig. 1. Résultats du modèle d’irradiation de [31]. Abondances produites par irradiation de , , , , et en adoptant une chimie chondritique et les paramètres spectraux et /. Les abondances (relativement aux isotopes stables respectifs) sont normalisées aux valeurs observées dans les météorites et mentionnées dans le Tableau 1. Adapté de [31].
The decoupling of and observed in some rare hibonite-bearing CAIs [49] can be accounted for by irradiation during gradual events instead of impulsive events [31]. Because gradual events have shallower energy spectra than impulsive events, they can overproduce relative to . In the contemporary Sun, gradual events are times less frequent than impulsive events, in agreement with the observation that hibonite-rich CAIs are rare.
Uncertainties of the model arise mainly from a poor knowledge of the nuclear cross sections, especially for the channel. Many of the cross sections used in [44] and [26,31] are the result of nuclear fragmentation codes. To reduce uncertainties in the cross section knowledge, nuclear physicists based at Orsay have undertaken a program of measurements of -induced cross sections [18,71]. Fitoussi et al. [18] found that the experimental cross section is a factor of lower than the estimate of [44], within the range of the announced uncertainties. Compared to what is observed in the contemporary Sun, the irradiation model also requires larger / ratios [72]. It was also recently argued that irradiation models do not produce enough to contaminate the whole accretionary disk [12], although observed heterogeneities in the distribution in the disk [28] indicate that it is not necessary for irradiation models to synthesize a minimum solar nebula of .
4 X-ray evidence for protoplanetary disk irradiation
The variable, intense and ubiquitous X-ray activity of young, solar-like stars is indicative of the acceleration of high-energy particles that have the possibility to irradiate gas and dust from the protoplanetary disk [78]. Although there is no direct evidence for high-energy particles irradiation, there is a growing evidence for X-ray irradiation of the disk dust.
T Tauri stars are low-mass (–2 M⊙) young stars, of effective temperatures 3000–4000 K, aged a few million years. Their internal structure is entirely convective. They have been known for a long time to be highly variable in the optical domain, and show strong chromospheric lines, like the Sun. Thus it has been long suspected that T Tauri stars are, like the Sun itself, the seat of magnetic activity (starspots, …), the magnetic fields resulting from convection-induced dynamo. With the advent of X-ray satellites in the late 1970s, the first cases of X-ray emission by T Tauri stars were immediately reported in nearby star-forming regions (Taurus, Ophiuchus, etc.).
In parallel, near- to mid-IR observations, both from the ground and in space, revealed that a significant fraction of T Tauri stars displayed an IR excess, i.e., were more luminous in IR, sometimes by a large factor, than the black-body emission of their photospheres. Using a variety of arguments, it was possible to explain this excess in terms of extended circumstellar disks, with a temperature law decreasing outwards. Direct imaging of young stars, in star forming regions like Orion, proved the existence of protoplanetary disks around these stars, consistent with the laplacian view of the solar system. These disks form the reservoir out of which matter falls onto the star and makes it grow.
It is now observationally proven that all T Tauri stars, whether of not they are surrounded by circumstellar disks, and for all masses, are X-ray emitters [17,21]. About 30 star-forming regions have been observed in X-rays, and they all show this same result. The X-rays are generally seen in the form of hour-long, solar-like flares, and this is interpreted as heating from reconnection of large magnetic loops (up to several stellar radii in a few cases, see, e.g., [15]). The general properties of the X-ray emission can be summarized as: the X-ray emission from T Tauri stars is qualitatively ‘solar-like’, and is a proxy for magnetic fields. It is true that X-rays from accretion shocks onto the star have been also observed, but these cases concern only a handful of stars so far, which are presumably in a state of low magnetic activity (e.g., [62]), compared with thousands of T Tauri stars showing the same magnetic activity in the form of X-ray flares (e.g., [21,32]).
Quantitatively, however, the X-ray luminosity, scaled to the stellar bolometric luminosity (the ratio) reaches levels several orders of magnitude above the solar X-ray luminosity: up to , but with a large dispersion. In absolute terms erg s−1, the dispersion being one or even two orders of magnitude either way. The dispersion is partly explained by a dependence on mass and age (e.g., [78]), and is much larger than that due to flare variability alone. The X-ray plasma generally displays two temperatures, a dominant hard component keV, attributed to magnetic activity, and a fainter soft component, of unclear origin but perhaps linked with accretion or shocks in a jet funnel close to the star (e.g., [34]).
For T Tauri stars surrounded by circumstellar disks, such high X-ray luminosities suggest the possibility to directly observe irradiation effects (Fig. 2). Glassgold et al. [24] were the first to compute the ionization effects of X-rays. They adopted a model in which the X-rays were emitted at high stellar latitudes (‘lamppost irradiation’), and the disk was ‘flared’, i.e. geometrically thicker far from the central star because of the decrease of the local gravity. The result was that, except in the equatorial plane where the disk density was highest, resulting in a column density absorbing the X-rays up to cm−2, the keV X-rays effectively ionized the gas out to large distances (several 10 AU). However, at higher altitudes above the disk plane, the X-rays, having a longer mean free path because of the reduced density, not only ionize the gas but also warm it, to temperatures as high as 3000–4000 K. The existence of such a warm, tenuous ‘atmosphere’ above a cold disk (typically K or less) offers a nice alternative explanation to the observations of CO overtone near-IR bands in T Tauri stars with disks [23].
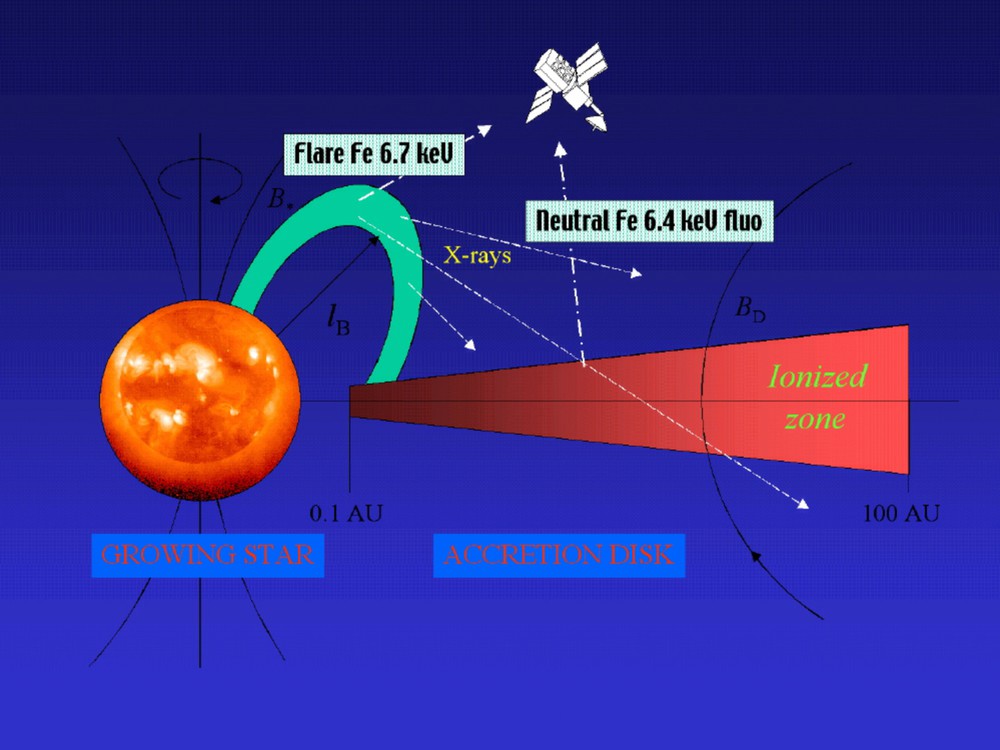
Generic sketch of the irradiation environment of circumstellar disks in protostars and T Tauri stars. The young star is magnetically active (as seen ubiquitously in X-ray observations). Based on the solar analogy, the X-ray emitting models are based on plasma confinement by large magnetic loops (height up to 1–2 stellar radii), either on the star itself or sometimes connecting the star and the disk. The magnetic loops sit at intermediate latitudes, allowing X-ray irradiation in a ‘lamppost’ fashion, out to large distances from the central star (10–20 AU). Among various irradiation effects, two have been directly observed in T Tauri stars: the fluorescence Fe K line at 6.4 keV (as shown here), and very recently the NeII 12.8 m line, as predicted by X-ray irradiation models. See text for details.
Fig. 2. Illustration schématique de l’irradiation des disques circumstellaires autour des protoétoiles et des étoiles TTauri. La jeune étoile est magnétiquement active, comme l’indiquent les observations dans le domaine des rayons X. Les modèles d’émission de rayons X, en analogie avec le soleil contemporain, sont basés sur le confinement du plasma par de grandes boucles magnétiques (jusqu’à 1 à 2 rayons stellaires), attachées à l’étoile elle-même ou parfois connectant l’étoile et le disque. Les boucles magnétiques se trouvent à des latitudes moyennes, permettant une irradiation de type «réverbère» jusqu’à des distances éloignées de l’étoile centrale (10 à 20 AU). Parmi les effets de l’irradiation observés autour des étoiles jeunes, on trouve la florescence de la ligne K du fer à 6,4 keV (illustré ici), et la ligne NeII du néon à 12,8 m. Cette dernière observation avait été prédite par les modèles d’irradiation (voir texte).
More recently, Glassgold and collaborators have explored further atomic ionization effects in disk atmospheres. They have shown, in particular, that X-ray irradiation would ionize neon out to 20–30 AU, with the emission of the NeII line at 12.81 m [22]. This line has now been seen in a number of T Tauri stars, consistent with theoretical predictions, by Spitzer, demonstrating the reality of a direct X-ray irradiation effect on disk atmospheres. This research is continuing with other atomic X-ray ionization diagnostics (sulfur, oxygen, etc.) that may be observable in the MIR and FIR ranges in the near future (SOFIA, Herschel).
Another, purely X-ray evidence for deeper disk irradiation comes from observations of the Fe K fluorescence line at 6.4 keV in a number of T Tauri stars with disks [15,73]. Here, the incoming X-rays, either more or less continuous from coronal emission, or more efficiently after a strong flare, induce a photoelectric effect directly on the ground-level electrons; the subsequent rearrangement of the upper levels by cascade, of Fe in particular, because it normally radiates at 6.7 keV, results in the emission of a 6.4 keV fluorescence photon. This effect had been predicted long ago [20], and is frequently observed in AGNs (‘active galactic nuclei’, which contain a supermassive black hole at their center). There are, however, geometric conditions involved, and the effect is best seen when the disk plane is within a few degrees of the plane of the sky (star viewed ‘pole-on’). This has a low statistical probability of occurring, so only a handful of sources displaying this fluorescence line have been discovered so far.
Even less numerous are T Tauri stars which show both the NeII IR line and the Fe K 6.4 keV fluorescence line (Ophiuchus cloud, Flaccomio et al., personal communication), but as IR and X-ray surveys of star-forming regions continue, the number of combined Ne–Fe detections should increase.
In any case, given the huge number of T Tauri stars with disks now known, which have distinctive common X-ray and IR properties, the fact that a number of them already show evidence for NeII 12.8 m or for FeK 6.4 keV emission, or even both, proves beyond doubt the reality, and ubiquity, of disk irradiation by X-rays. This is a solid, albeit indirect, indication that the early solar system must have been similarly irradiated.
5 The initial abundance and origin of
The initial value of in the nascent solar system is still uncertain. The / ratio of might be a too high estimate of the initial content in the solar system. Most measurements on chondrules from primitive meteorites including Semarkona yield measured / ratios between 2 and [58,70], while one measurement from a Semarkona troilite (FeS) gives / [54]. High / initial ratios in troilite might be due to Fe-Ni redistribution in the sulfides during later alteration processes [33]. It is difficult to understand how troilite would have formed with a higher initial / ratio than chondrules in the same meteorite. Ni isotopes measurements in CAIs are challenging. An upper limit on the / ratio of was given by Birck and Lugmair [3] for an Allende CAI having a Fe/Ni ratio of . A two point internal isochron for an Allende CAI measured by Quitté et al. [58] gives an initial ratio / of . Some researchers claim that the initial / ratio could have been as low as [69].
The low part of the range is compatible with a galactic background origin for . The present average galactic value of the / ratio has been estimated to be [11]. Recent measurements of -ray lines in the interstellar medium with the Integral satellite have yielded a ratio / [76]. Using an elemental ratio / [47] gives an average galactic value for / of . This latter value is identical to the lower value of the early solar system estimate inferred from meteorites. Even if the / ratio was as high as [58], fluctuations around the average value could easily explain the presence of due to a galactic background in the solar system. It is important to note that this estimate is that of the present interstellar medium. It might have been significantly different in the interstellar medium 4.4 Gyr ago. Such calculations only show that if the initial solar system / ratio was only a few times , the need for a single supernova vanishes and leaves plenty of room for its galactic origin.
Acknowledgments
We thank Anders Meibom for his careful reading of the manuscript and Jean Duprat for his thorough review.