1 Introduction
Atmospheric CO2 is a greenhouse gas likely responsible for major climate changes during the history of the Earth. Rapid CO2 increase is also one of the main factors responsible for the recent global warming (IPCC, 2013). In order to quantify and calibrate the sensitivity of global warming to changes in atmospheric CO2 concentrations, past pCO2 variations need to be determined and compared with contemporaneous temperature estimates. For the last 800,000 years, this has been possible using direct atmospheric pCO2 measurements and temperature estimates from ice cores (e.g., Siegenthaler et al., 2005). For longer timescales, proxies of CO2 sources (i.e. volcanic activity) and sinks (continental weathering) may be used for modeling these variations (e.g., Godderis and Veizer, 2000). Biological proxies such as stomatal indices and carbon isotope compositions (δ13C) of marine organic matter (notably of alkenones, which represent fossil coccolithophores or organic material from diatom frustules) can also provide estimates of atmospheric CO2 (Kürschner et al., 2008; Pagani et al., 2005). However, stomata fossils are rare and phytoplankton δ13C can be influenced by unrelated factors such as cell size, paleoproductivity and CO2 uptake mechanisms (Laws et al., 2001). Other promising approaches for reconstructing surface-ocean carbonate chemistry over the last ∼100 Ma use proxies for paleo-pH or for paleo-carbonate ion (CO32–) concentrations. Boron isotope compositions (δ11B) (Rollion-Bard et al., 2011) of foraminifera shells have been shown to be a proxy of ocean paleo-pH (e.g., Foster, 2008; Pearson and Palmer, 2000; Pearson et al., 2009; Sanyal et al., 1995; Seki et al., 2010). However, pH alone is not sufficient to calculate the complete ocean carbonate chemistry since two parameters (typically pH and alkalinity or DIC) are needed in order to calculate the CO2(aq) from which atmospheric pCO2 can be estimated. Previous studies generally assumed a conservative ratio between alkalinity and salinity at the million-year scale. However, this approach may result in significant uncertainties in the final pCO2 estimates because the alkalinity–salinity relationship of the past ocean could have varied. More recently it was suggested that B/Ca ratio in benthic foraminifera may be used to estimate ΔCO32– (i.e. the deviation of seawater from calcite saturation; e.g., Rae et al., 2011; Yu and Elderfield, 2007; Yu et al., 2010), but both δ11B and B/Ca are associated with the complex variability of boron speciation and its incorporation into calcite crystals (Klochko et al., 2009). In this study, we describe a new foraminiferal proxy for DIC in the past ocean: the ratio between 7Li and 6Li isotopes, expressed as δ7Li (δ7Li = 1000 × [7Li/6Li/7Li/6LiLSVEC–1]), in their calcite shells, which can provide additional constraints for the quantification of the past carbonate system in the ocean and past atmospheric CO2.
Present-day seawater is homogenous with respect to Li concentration (175 μg/L) and isotopic composition (δ7Li = 31.2 ‰, Millot et al., 2004). This is explained by the long residence time of lithium in the ocean relative to the oceanic water mixing time (see review in Burton and Vigier, 2011; Tomascak, 2004). Li isotopes fractionate significantly during low and high temperature geochemical processes, in particular during weathering of silicate rocks (Schmitt et al., 2012). Waters are systematically enriched in the heavy isotope (7Li) relative to fresh rocks and secondary phases (e.g., Huh et al., 2001; Vigier et al., 2008). The inverse trend between river δ7Li and silicate weathering rates, highlighted by most watershed studies (Kisakurek et al., 2004; Pogge von Strandmann et al., 2006; Vigier et al., 2009), could make Li isotope composition of past oceans a proxy of variation of continental weathering through time, and therefore of corresponding atmospheric CO2 consumption. Thus, Hathorne and James (2006) and Misra and Froelich (2012) have interpreted foraminifera δ7Li in terms of variation of continental weathering for the period 0–18 Ma and 0–70 Ma, respectively. In addition, von Strandmann et al. (2013) interpreted δ7Li variation measured in OAE2 (Ocean Anoxic Event, 93.5 Ma ago) carbonates by an increased weathering rate. Carbonates are only a minor sink of oceanic lithium. However, depending on how foraminifera record the Li isotope composition of the ocean at the time of their shell formation, they could provide precious information either:
- • on variations of the main ocean Li sources (hydrothermal activity and continental flux) and sinks (low temperature oceanic crust weathering and clay formation) or;
- • on environmental conditions at the time of their formation.
The latter can be envisioned if the dependence of Li isotope fractionation on environmental parameters such as ocean temperature (T), pH or DIC during foraminiferal calcification is known. For periods longer than 1 Ma (the oceanic Li residence time), both the variations of oceanic δ7Li and of environmental conditions may influence the foraminifera Li/Ca and Li isotope compositions. In order to determine separately the role of each of these parameters on Li isotope fractionation, benthic foraminifera of the genus Amphistegina were grown at various temperatures (18 °C, 21 °C, 24 °C, 33 °C), various pH (7.90, 8.10, 8.20, 8.45) with constant DIC, and various DIC concentrations (1.0, 1.5, 2.0, 2.5, 3.0 mmol·kg−1) at constant pH and T.
2 Methods
Amphistegina is a large epibenthic symbiont-bearing, calcitic-radial perforate genus, which is abundant in coral reef environments. Before the experiments, live Amphistegina specimens were collected from Halophila leaves at a depth of ∼8 m or from turf algae on stones in the northern Gulf of Eilat (Red Sea) in February 2005. Two different species were used for the following experiments: A. lessonii for temperature experiments and A. lobifera for pH and DIC experiments. These two species present the same physical characteristics (knob and keel areas) and seem to have the same geochemical behavior (see, for example, Segev and Erez, 2006). Live specimens were then placed in sealed Erlenmeyer bottles filled with natural seawater modified in order to reach the target values of pH and DIC (see below and Rollion-Bard et al., 2008). After the experiments, Li isotopes and Li/Ca ratios were measured in the foraminifera shells using the ims 1270 ion microprobe, following a technique previously developed (Rollion-Bard et al., 2009; Vigier et al., 2007).
2.1 Cultures performed at various temperatures
The temperature experiments were carried out with live Amphistegina lessonii, collected from turf algae on stones in the coral reef environment in the Gulf of Eilat, Israel. Uniform populations of ∼400 μm of diameter were placed in sealed 60-mL glass stoppered Erlenmeyer bottles filled with natural seawater. The natural seawater was surface-nutrient depleted-water from the Gulf of Eilat. This seawater has an initial salinity of 40.7‰ that was adjusted to 35‰ (by adding distilled water). The Erlenmeyer bottles were immersed for 2–3 months in temperature-controlled baths (at 18, 21, 24, 27, 30, and 33 °C respectively), keeping the assigned temperatures to ±0.1 °C. Water in each bottle was replaced twice a week with fresh seawater. The pH in these experiments ranged between 8.1 and 8.2 with lower pH values at the higher temperatures due to faster calcification. All bottles received the same amount of natural light (∼ 10–15 μmol photons m−2 s−1) and were examined daily for the development of algae. The foraminifera in these experiments increased their weight from 50 μg/individual to between 95 and 135 μg/individual, with optimal growth at 27 °C.
2.2 Cultures performed at various pH
Amphistegina lobifera, collected similarly as above, were used for the cultures undertaken at various pH. The open Gulf of Eilat water used in the experiments has an average DIC of 2070 ± 10 μmol·kg−1 (Silverman et al., 2007), and the original alkalinity is roughly 2500 μmol·kg−1. These waters were diluted to a salinity of 35 ‰ with double-distilled water (DDW), and were boiled to remove CO2. The DIC and the original alkalinity were thus diluted by the salinity factor of 35/40.7 (as explained above); hence the DIC was roughly 1780 μmol·kg−1. These experiments were done at 24 ± 0.1 °C and the carbonate chemistry was modified by keeping the DIC constant, while the pH was lowered with HCl or raised with freshly made NaOH. The seawater reservoirs were kept out of contact with the atmosphere in plastic collapsible containers. Water was pumped with a peristaltic pump from the containers into tightly capped 60-mL Erlenmeyer flasks containing the foraminifera at flow rates between 160 and 180 mL/day. The cumulative alkalinity of the out-flowing water was measured every three days and the calcification of the foraminifera was estimated from the alkalinity difference between the reservoir and the outflow. In addition, the weight increase of the foraminifera was estimated from the final weight of the individuals compared to that of the initial control group. The weight increased on average by a factor of 5 (from 18 μg/individual to about 100 μg/individual). The different pH values for this experiment were 7.90, 8.10, 8.20 and 8.45 ± 0.10 (NBS scale).
2.3 Cultures performed at various Dissolved Inorganic Carbon contents
Amphistegina lobifera were used for the cultures undertaken at various DIC. The diluted Gulf of Eilat seawater was acidified with HCl to pH 2.8 and air bubbled for 12 h to get rid of most of the dissolved inorganic carbon. Then, NaHCO3 was added to get final concentrations of DIC of 1.0, 1.5, 2.0, 2.5, and 3.0 mmol·kg−1, respectively. For comparison, the DIC range in the ocean is 1.8–2.4 mmol·kg−1. The pH was adjusted with freshly prepared 1 N NaOH and 1 N HCl in order to obtain values of 8.15 for all of these waters with different DIC. The pH values ended up with a small variability of ≈ 0.01 pH units. The reagents used were the same as those for varying the pH in the previous experiments except for the NaHCO3 (section 2.2). The water reservoirs were isolated from the atmosphere inside collapsible plastic reservoirs connected to a peristaltic pump that pumped the diluted modified seawater solutions through each Erlenmeyer flask where the foraminifera were growing. The water coming out of the Erlenmeyer was collected and weighted (every 2 or 3 days) and measured for its alkalinity. Occasionally, we also measured oxygen and pH in the water coming out of the Erlenmeyer flasks. The alkalinities were close to the original ones, but always slightly lower (because of the foraminiferal calcification). The pH of the outcoming waters was slightly different from that in the reservoir because of the metabolism of the foraminifera.
2.4 Li isotope and Li/Ca measurements in foraminifera tests
After the experiment, the cultured foraminifera were rinsed with double-distilled water (DDW) and dried overnight at 50 °C. Specimens were then placed in dilute sodium hypochlorite (1:3) for 7 h followed by several DDW rinses and overnight drying at 50 °C. Before analyses, samples were embedded in epoxy sections, polished using a diamond paste down to 1 μm and then coated with gold. Samples were put horizontal and were polished until the appearance of the top part of the knob (Rollion-Bard et al., 2008). Thus, only the sample part grown under laboratory conditions was analyzed with the ion probe.
Li isotope analyses and Li/Ca ratios were performed in situ with the ims 1270 ion probe at CRPG (Nancy, France). The analytical settings in this study are the same as those described in Vigier et al. (2007) and in Rollion-Bard et al. (2009). Briefly, the lithium isotopic compositions and Li/Ca ratios were measured using a 20- to 30-μm 16O primary beam of ∼50 nA. The mass resolving power (MRP) was set at ∼3000, with the energy slit well centered and fully opened. A single Li isotope analysis consists of peak switching on masses 6 and 7, which are successively counted on an electron multiplier. After 2 min of presputtering, each run lasts about 20 min (40 to 60 cycles of 12 s and 10 s, respectively). The background is measured every cycle for 2 s on mass 5.5. The instrumental mass fractionation (IMF) and the reproducibility of the isotopic analyses were calculated from repeated measurements of the CAL-HTP calcite reference material that was also analyzed by MC–ICPMS for Li isotopes (δ7Li = 13 ± 0.5‰, 2σ). The isotopic homogeneity of this sample was confirmed by multiple ion microprobe measurements along profiles (Vigier et al., 2007), and the reproducibility is better than 1‰ (1σ, n = 10).
For Li/Ca ratios, the analyses consist of peak switching on masses 7 (7Li+) and 44 (44Ca+), which are successively measured on an electron multiplier and on a Faraday cup. The Li content was also measured in a glass standard (384 ppm Li, and 0.57 W.% CaO). No difference in the yield was observed between carbonate and glass standards. Backgrounds are measured every cycle for 2 s on masses 5.5 and 43.6 to ensure magnet stability. Li/Ca was analyzed in the same spot as δ7Li. External (spot-to-spot) reproducibility for Li/Ca ratios was determined from repeated measurements of the glass reference material and was better than 1% (1σ). The total precision for each Li/Ca analysis includes external reproducibility and internal precision.
3 Results
3.1 Assessing T and pH controls
Li/Ca and δ7Li data are reported in Tables 1, 2 and 3. δ7Li measured in the Amphistegina shells display no significant variation as a function of temperature and pH (Fig. 1a and b). For both sets of experiments, mean δ7Li values are close to the δ7Li of seawater (30.0 ± 0.9‰ and 29.8 ± 0.6‰ respectively, Fig. 1). This strongly suggests little isotope fractionation relative to the seawater – within uncertainty – for the given set of conditions. However, this is significantly different from the fractionation of Li isotopes observed during experimental growth of inorganic calcite, and strongly suggests a vital effect in favor of the heavy 7Li isotope (Δ7Li calcite–solution = –3‰ to –9‰, see Marriott et al., 2004a, 2004b). These results are also consistent with part of the published core top foraminifera data, which exhibit the same isotope compositions as seawater. However, pH and T cannot explain the significant range of δ7Li values measured in core top planktonic species (e.g., Hathorne and James, 2006; Misra and Froelich, 2012; Vigier et al., 2007).
Results for the series of cultures performed at constant temperatures (24 °C), constant DIC (1.8 mmol·kg−1) and different pH values.
pH | Li/Ca (μmol·mol−1) | δ7Li (‰) |
7.9 | 30.2 | |
7.9 | 29.0 | |
7.9 | 30.2 | |
7.9 | 14.1 | 29.4 |
7.9 | 12.7 | 31.1 |
7.9 | 14.8 | 29.1 |
7.9 | 13.3 | 29.5 |
8.1 | 29.8 | |
8.2 | 15.5 | 29.8 |
8.2 | 29.9 | |
8.45 | 29.8 | |
8.45 | 13.5 | 29.8 |
8.45 | 12.9 | 29.4 |
Results for the series of cultures performed at constant pH (8.15), constant DIC (1.8 mmmol·kg−1) and different temperatures.
Temperature (°C) | Li/Ca (μmol·mol−1) | δ7Li (‰) |
18 | 16.3 | 29.8 |
18 | 17.4 | 30.0 |
18 | 15.8 | 30.7 |
21 | 18.3 | 30.9 |
24 | 17.6 | 28.7 |
24 | 16.8 | 29.6 |
24 | 14.5 | 30.3 |
33 | 14.4 | 28.3 |
33 | 15.9 | 30.4 |
33 | 17.6 | 31.1 |
33 | 15.9 | 29.8 |
Results for the series of cultures performed at constant temperature (24 °C) and different DIC.
DIC mmol·kg−1 | Li/Ca (μmol·mol−1) | δ7Li (‰) |
1.0 | 19.7 | 21.6 |
1.5 | 15.3 | 23.2 |
1.5 | 14.3 | 26.3 |
1.5 | 15.7 | 27.2 |
2.0 | 14.4 | 32.7 |
2.0 | 16.5 | 31.2 |
2.0 | 14.4 | 31.2 |
2.0 | 32.1 | |
2.5 | 3.6 | 41.9 |
2.5 | 3.4 | 40.7 |
3.0 | 5.9 | 37.7 |
3.0 | 6.0 | 37.7 |
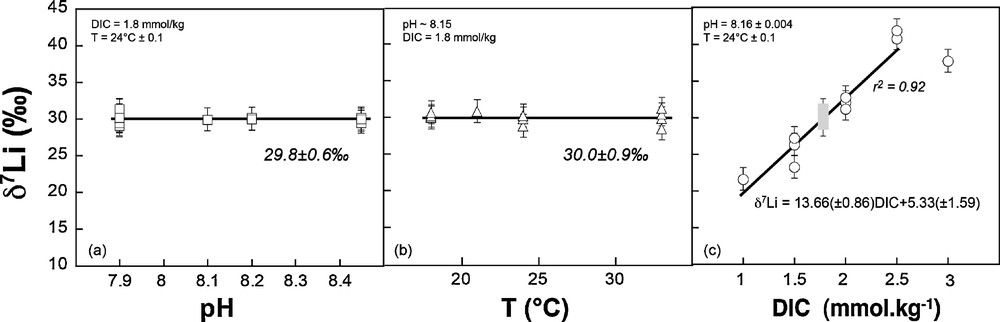
δ7Li measured in Amphistegina tests as a function of culture pH (a), culture temperature (b) and culture dissolved inorganic carbon (c) (see Tables 1–3). The linear regression and corresponding errors on the slope and intercept shown in Fig. 1c were obtained using Axum50 software. The grey rectangle corresponds to the range given by Amphistegina grown at various T and pH. The linear regression does not include the point obtained at high DIC (3 mmol·kg−1), and is therefore valid for DIC ranging between 1 and 2.5 mmol·kg−1.
Similarly, Li/Ca measured in the same tests exhibit no dependency on T and pH, with mean values of 16.4 ± 1.3 μmol·mol−1 and 13.8 ± 2 μmol·mol−1, respectively (Tables 1 and 2, Fig. 2). For comparison, the Li/Ca ratio of seawater is currently 2365 μmol·mol−1. The lack of correlation with temperature is in agreement with studies of core top planktonic species (Hathorne and James, 2006). However, Lear and Rosenthal (2006), Lear et al. (2010), and Bryan and Marchitto (2008) have suggested that concentration in CO32– could control the Li/Ca ratio of benthic foraminifera. Here, no significant influence of the pH is seen on both Li/Ca and δ7Li values. In this experiment performed at various pHs, but constant DIC and T, the CO32– ion concentration changed by 0.4 mmol·kg−1 and this did not affect the Li/Ca ratio nor the δ7Li. We can therefore reasonably rule out a key role of this parameter, at least for Amphistegina species. Similarly, Hathorne et al. (2009) show no significant influence of the carbonate ion concentration on Li/Ca measured in situ by LA ICPMS in the calcitic tests of two different planktonic species.

Li/Ca measured in Amphistegina tests as a function of culture pH (open symbols) (B), culture temperature (black symbols) (A) and DIC (in grey circles) (C). The average of all values measured at various pH and T is 15.4 ± 1.7 μmol·mol−1 (grey line on A and B).
3.2 Assessing DIC control
In contrast to temperature and pH (or CO32–), both δ7Li and Li/Ca ratio exhibit large variability and good linear correlations with the solution DIC (Figs. 1 and 2). Amphistegina δ7Li values positively and linearly correlate with DIC. There is a strong dependency on DIC, with 20‰ differences between foraminifera tests cultured at 1.0 mmol·kg−1 DIC and at 2.5 mmol·kg−1 DIC. In parallel, Li/Ca ratios increase from ∼4 to ∼20 μmol·mol−1 as DIC decreases from 2.5 to 1.0 mmol·kg−1 (Fig. 2, Table 3). For both δ7Li and Li/Ca the experimental data corresponding to the highest DIC value (3.0 mmol·kg−1) falls off of these linear trends. We can rule out a potential contamination from the reagents used in the DIC experiments, for several reasons. First, we used the same reagents as for pH experiments, except HCO3–. We added HCO3– in order to obtain the required DIC value, but if this procedure would have resulted in an increase of solution Li content, then the Li/Ca ratio in the shells should be positively correlated with DIC. The actual trend is the opposite (see Fig. 3). Moreover, since the same HCO3– reagent was added for both low- and high-DIC experiments, the variation of δ7Li going from values below the seawater Li isotope composition to values higher than seawater δ7Li could not be explained by the addition of this reagent. Thus, the increase in the δ7Li and decrease in the Li/Ca ratios must be caused by the DIC increase and related mechanism of calcite precipitation.
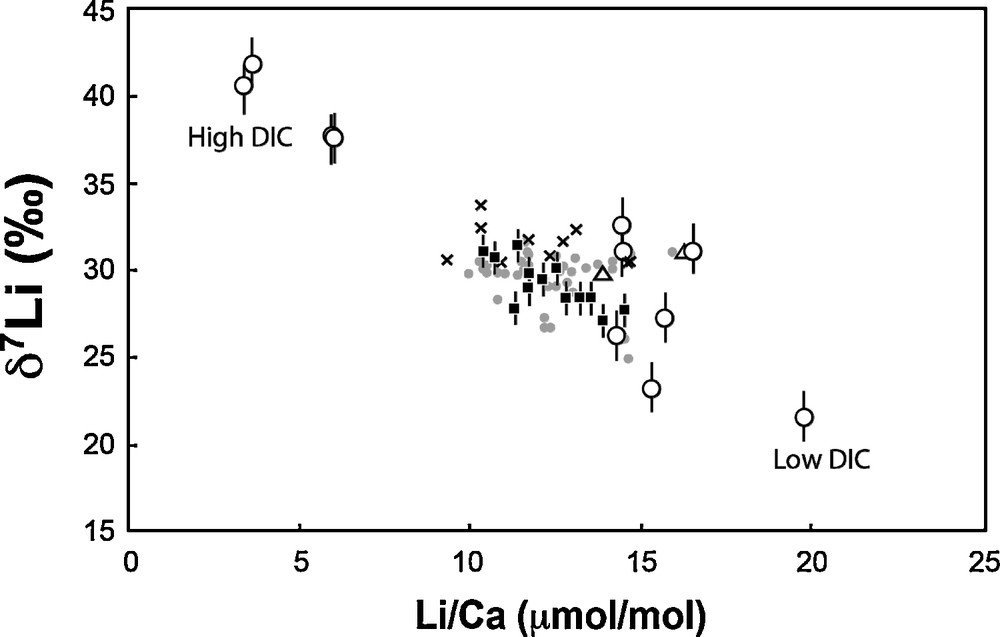
δ7Li versus Li/Ca measured in Amphistegina cultured at various DIC (white circles). The white triangles correspond to the average values given by the Amphistegina cultured at various T and pH (see Fig. 1a and b). On this plot, are also reported the published data for various species of planktonic foraminifera: squares, core top from Hathorne and James (2006); grey circles, core top from Misra and Froelich, (2012); crosses, Glacial/Interglacial from Hall et al. (2005).
4 Discussion
4.1 Biological control on foraminifera δ7Li
Our cultures indicate the key role of the concentration of dissolved inorganic carbon on the Li isotope composition and on the Li/Ca ratio of Amphistegina tests. Figs.1c and 2 show that, for given T and pH values, at low DIC, the calcite Li/Ca ratio is high and the δ7Li value is low. As described previously, we first rule out a potential influence of the carbonate ion (CO32–) concentration. The foraminifera grown at various pH with constant DIC experienced significant CO32– variability, yet their Li/Ca and Li isotope compositions remained constant (Fig. 1a). The same argument can be made with respect to alkalinity: the range of alkalinities in the pH experiments was significant (0.4 mmol·kg−1) and again the δ7Li and Li/Ca were similar, within uncertainties. One possibility to explain the dependence of Amphistegina Li/Ca and δ7Li on DIC concentrations is that it may be related to the physiology of calcification in perforated foraminifera (Bentov et al., 2009, Erez, 2003). In previous studies, it has been shown that seawater vacuoles provide the fluid from which the shell is precipitated (e.g., Erez, 2003). In order to precipitate CaCO3 from these seawater vacuoles, the foraminifera have to concentrate DIC until the CO32– content matches (at least partially) the Ca2+ concentration in the seawater vacuoles, which is between 10 and 11 mM. This internal carbon pool has been thoroughly described using 14C pulse-chase experiments with A. lobifera (ter Kuile and Erez, 1987, 1988; ter Kuile et al., 1989). In order to concentrate DIC in the vacuoles, the foraminifera elevate their pH and the DIC is increased by diffusion of CO2(aq) from the cytosol into the vacuolated seawater (Bentov et al., 2009, Erez, 2003). Elevation of pH in the vacuoles and the calcifying fluid may be achieved through several ways. For example, Ca-ATPase has often been suggested as one potential candidate in corals (e.g., Al-Horani et al., 2003; McConnaughey, 1989; Venn et al., 2013). However, there are other possibilities for such proton transporters, which are protein complexes that transport protons against the concentration gradient (note that the cytosol in which the vacuoles are residing has a low pH of about 7.2–7.4). Usually such processes require ATP, as an energy source, or utilize the cellular electrochemical gradient to achieve the needed result. We suggest that in foraminifera, this proton transport is achieved by an involvement of a Na/proton exchanger (antiporter) or by another pathway in which Na (and Li) ions are transported into the vacuole while protons are transported out into the cytosol. We propose a coupling between Na and Li because Li can often replace Na during such trans-membrane processes, due to similar chemical behavior and charge (Parker, 1986). It is interesting to note the non-dependency of Li and δ7Li on the experimental temperature, suggesting that Li and Mg behaviors are decoupled, despite similar ionic radii (assuming that Mg/Ca and T are correlated). This indicates that the ion charge is an important parameter in biological activity (in contrast to abiotic reactions where Li+ and Mg2+ are often coupled; e.g., Huh et al., 2001; Vigier et al., 2008). Our observations may therefore be explained as follows: when the solution DIC is low, the activity of the Na/proton exchanger is intensive in order to elevate the pH, the alkalinity, and therefore to accumulate DIC in the vacuoles. While this is happening, Li follows Na and accumulates in the vacuoles. Under these conditions, the lighter isotope (6Li) may be preferentially transported into the vacuoles, which would explain the high Li/Ca ratio associated with the low δ7Li values (Fig. 3). When CaCO3 is precipitated from modified seawater, it records its Li concentration and its δ7Li value. Further speculations on this mechanism are perhaps too early, but it explains well our experimental observations and is in good agreement with the models suggested for foraminiferal calcification (Bentov and Erez, 2005; Bentov et al., 2009; Erez, 2003).
When DIC is high, calcite δ7Li is also higher (higher than seawater value, 31‰) and Li/Ca is lower (Fig. 3). Published δ7Li values for core-top foraminifera tests higher than 31‰ (seawater) are rare, but exist (see, e.g., Hall et al., 2005; Kosler et al., 2001). Actually, these values are still consistent with a vital effect in favor of the heavy 7Li isotope, as underlined in section 3.1 and shown by T and pH experiments (Fig. 1a and b), compared to inorganic calcite (see section 3.1). Under high DIC conditions, we can speculate that there is no need to increase the DIC in order to calcify, and therefore, the high δ7Li observed in the Amphistegina tests cultured in these conditions likely reflects the isotope composition of the solution at the calcifying site. The reaction responsible for this increase may also be either kinetic or associated with a Rayleigh-type isotope fractionation occurring in the cytosol with preferential release of the light 6Li isotope, and decrease in Li/Ca ratio. A more refined biological study of the mechanisms occurring at high DIC is underway.
4.2 Applications to natural conditions
Amphistegina are symbiont-bearing foraminifera, belonging to the calcitic-radial perforate group, and thus are thought to precipitate their calcite using the same mechanisms as most planktonic foraminifera (Erez, 2003). This was illustrated by boron isotope ratios measured on cultured Amphistegina tests, using SIMS, which can be explained by a pH range similar to the one measured by pH microsensors in living planktonic Globigerinoides sacculifer (Jorgensen et al., 1985; Rollion-Bard and Erez, 2010). In order to test this for Li isotopes, we reported in Fig. 3 all the Li isotopes and concentration data published for various species of recent and core top planktonic foraminifera. The data plot along the trend defined by the Amphistegina cultured in this study. As a consequence, we consider that the trend between Amphistegina δ7Li and DIC may also be valid for the various planktonic foraminifera species studied thus far. It should therefore be possible to calculate the DIC at the time of the foraminifera test formation, using the linear regression of the experimental data points of Amphistegina (Fig. 1c). In addition, the DIC control evidenced in this study may partly explain the large published range of δ7Li measured for recent and sub-recent foraminifera (21.1–33.7‰, Hall et al., 2005; Hathorne and James, 2006; Marriott et al., 2004b; Misra and Froelich, 2012).
For short periods of time, such as for the last glacial/interglacial period, the ocean δ7Li has remained constant since the ocean Li residence time is larger than 1 Ma. However, foraminifera exhibit δ7Li variations during the last ∼40 ka (Hall et al., 2005). From 400 yr to 35.8 ka, the foraminifera δ7Li range is 30.4–33.7‰, which corresponds to a narrow DIC range (1.82–2.08 mmol·kg−1), using the empirical relationship determined experimentally. For this period of time, the pCO2 range is well known, since it is given by direct measurement of CO2 trapped in air inclusions analyzed in the Vostok ice cores (Monnin et al., 2001). Using the calculated DIC and the following equation:
(1) |
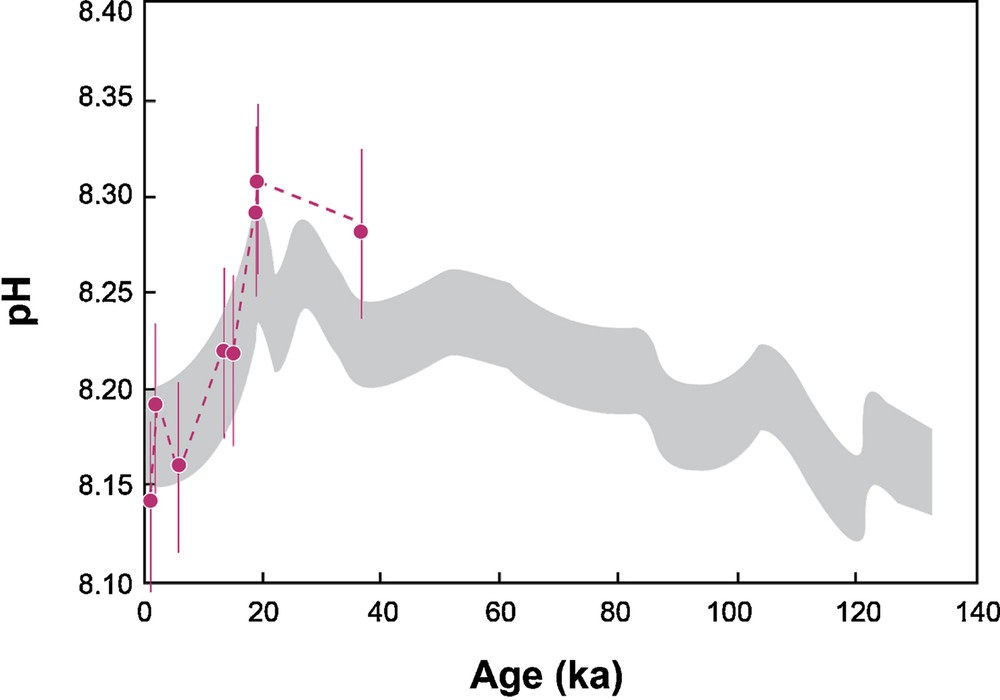
(Color online.) pH values (in pink) calculated from DIC using the δ7Li measured in foraminifera for the 0.4–35.8-ka period (Hall et al., 2005) and the pCO2 values taken from ice core (Monnin et al., 2001). The calculated pHs are compared to the pH variation determined by boron isotopes and B/Ca, shown in grey (Foster, 2008).
The 0–70 Ma record of δ7Li in foraminifera (Misra and Froelich, 2012) is also characterized by several periods of significant short-term variability (see Fig. 5b). This is particularly visible during the Miocene, between 11 Ma and 24 Ma (with, e.g., a 3.2‰ range of the δ7Li values between 14.25 Ma and 15 Ma). Since the Li residence time in the ocean is roughly 1 Ma, such rapid fluctuations cannot be explained by ocean variations due to changes in source or sink. Instead, our experimental results suggest that they can be related to changes of the Li isotope fractionation during foraminiferal growth, due to rapid variations of the DIC in seawater. We show that, for four critical periods, ranging between 16.5 Ma and 4.3 Ma, the foraminifera δ7Li and Li/Ca are linearly correlated and can be fitted by a similar slope as the one defined by the cultured Amphistegina and the core-top foraminifera (Fig. 3 and 5a). This strongly supports a DIC control on foraminiferal δ7Li at these times, which also correspond to critical periods of the Earth's history, i.e. the beginning of the Paleocene and the Mid-Miocene climatic optimum. The corresponding DIC variations range between 0.10 mmol·kg−1 in the Pliocene up to 0.22 mmol·kg−1 at the Miocene.

(Color online.) A. δ7Li versus Li/Ca for Amphistegina cultured with various DIC, and for core-top planktonic foraminifera (black circles: this study, Hathorne and James, 2006), and for 0–70 Ma foraminifera (grey circles and colored symbols, Misra and Froelich, 2012). Yellow triangles highlight all values displayed for the 3.15–5.5 Ma period, green diamonds for the 5.5–5.61 Ma period, blue diamonds for the 14.25–15 Ma period, and pink squares for the 16.1–17 Ma period; B. The 0–70 Ma foraminifera record of Misra and Froelich (2012) (grey circles) as a function of time. The periods highlighted in color in Fig. 5A are reported here using the same symbols (see text for more details). The corresponding maximum DIC variations (ΔDIC) have been calculated using the equation given in Fig. 1c, and assuming no variation of ocean δ7Li.
5 Conclusion
Using foraminifera cultures, we determined the influence of T, pH and DIC concentration on their calcite-seawater Li isotope fractionation and Li/Ca ratio. No significant influence of T and pH were observed, and the apparent lack of significant isotope fractionation suggests a biological control. A highly significant linear DIC-δ7Li relationship was observed as well as with Li/Ca ratio. These findings were used to re-interpret parts of published Cenozoic records in terms of DIC changes in the past ocean. The precision of this method still needs to be improved, but the first results are consistent – within uncertainties – with published past estimates of pCO2 and pH. Over short timescales, shorter than the ocean Li residence time (1 Ma), the Li isotope composition of foraminifera provides a new proxy of the ocean DIC, which, combined with other paleo-proxies of the carbonate system such as boron isotopes, may represent a great potential for reconstructing large and rapid variations of the past oceanic carbon cycle.
Acknowledgements
The authors thank the Editor François Chabaux, and the two reviewers, Franck Bassinot and Paul Tomascak for their constructive comments. Marc Chaussidon (CRPG, Nancy), Christian France-Lanord (CRPG, Nancy), Yehoshua Kolodny (University of Jerusalem), and Greg Ravizza (University of Hawaii) for fruitful discussions on an earlier version of the manuscript. Pete Burnard (CRPG) is thanked for English corrections. This project was funded by a regional grant (CNRS/Lorraine Region), by CNRS–INSU programs (SYSTER, INTERRVIE and LEFE-CYBER) and by the Israel Science Foundation (grants 551/10 and 870/05).