1 Introduction
In this article, we attempt to provide an overview of the activities of measurement of stratospheric ozone (and related species) organized in networks of ground-based measurements. Such activities started already around the middle of the 1920s and were devoted to increase the scientific knowledge and understanding of atmospheric ozone, driven by scientific curiosity. It is remarkable how important international (though at that time mainly European) scientific cooperation was. Within this cooperation, a network of scientists was set up by the International Ozone Commission (IO3C), organizing measurements and conferences that built the basis for international cooperation between ozone scientists still in place today (for a comprehensive description of the long history of IO3C, see Bojkov, 2010). A paradigm change occurred in ozone science in the early 1970s when anthropogenic ozone depletion became a new issue debated both in the science community and by the general public. At that time, the question of the reliability of the ozone measurements (overseen by the IO3C) became a high priority that led to the activities that are currently part of the Global Atmosphere Watch (GAW) program organized by the World Meteorological Organization (WMO). These ground-based measurements still provide the longest time series of stratospheric ozone, as several started in the late 1950s or even earlier. The history of the ozone monitoring networks is shortly described in Section 2; in Section 3, we describe the concept to ensure high quality in ozone measurements. In Section 4, we address experimental developments after implementation of the Montreal Protocol (MP) when requirements of ground-based monitoring became more challenging and a new network based on more modern instruments was established in the early 1990s, presently known as Network for the Detection of Atmospheric Composition Change (NDACC), which is described in Section 5. The ozone measurements over the South Pole are discussed in Section 6, and conclusions are presented in Section 7.
2 History prior to stratospheric ozone depletion
Fabry and Buisson (1921) were the first to provide reliable measurements of total (column) atmospheric ozone from Marseille in France. In the mid-1920s, Dobson (1968) constructed a new instrument, the Fery spectrophotometer, providing relatively easy measurements of total ozone. He was thereafter able to produce several such spectrophotometers (Dobson and Harrison, 1926). The signal was detected by photographic plates. These pioneering measurements allowed him and his collaborators to obtain information on the relationship between total ozone and atmospheric pressure at northern mid-latitudes, as well as the seasonal variation of column ozone that appeared to be different between the two hemispheres (Dobson, 1930).
In the next generation of sun spectrophotometers, generally known as Dobson spectrophotometers, photoelectric detection was used, leading to improved sensitivity. With this new instrument, ozone profile information can be derived from zenith sky measurements performed over a few hours starting prior to sunrise or extending over sunset (Fig. 1). This method is called the Umkehr method. It marked an important milestone in ozone science, namely indicating that the ozone partial pressure maximum occurs around 22 km at mid-latitudes (Götz et al., 1934).
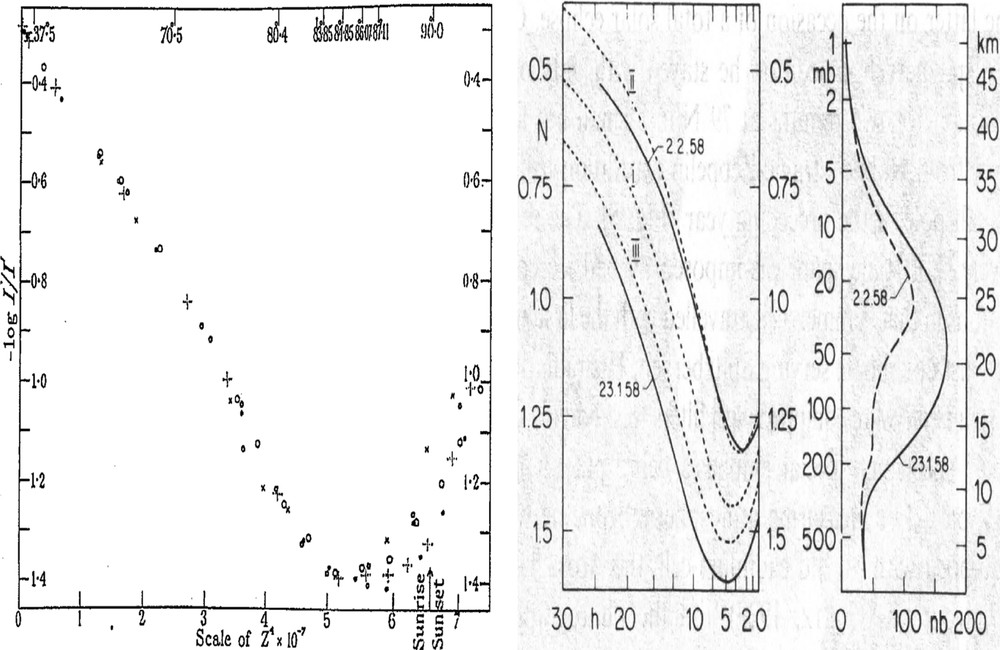
Ozone profile determination by the Dobson Umkehr method (Götz et al., 1934). The zenith sky measurements are performed for around 2–3 h to include sunrise or sunset. In the left panel, the signal (N-values) is shown as function of the fourth power of solar zenith angle (SZA) to illustrate the “Umkehr effect” occurring when the sun is close to the horizon. In the middle panel, the measurements (plotted as function of sun elevation above the horizon, in°) are depicted for two winter days of 1958 to show the retrieved ozone profiles (see right panel) plotted as ozone in partial pressure (in nbar) vs. altitude (pressure in mbar) (Dütsch, 1987).
A few years after World War II, photomultipliers could be used in Dobson instruments and the measurement technique for total ozone measurements was refined and coordinated. Total ozone measurements by Dobson spectrophotometers are based on wavelengths pair measurements using an optical wedge. Measurements using a single wavelength pair, however, can be perturbed by atmospheric aerosols as they absorb and scatter light in the wavelength region of 300 to 340 nm, in which range Dobson instruments are measuring the solar light. However, when using two wavelengths pairs of observations, the interference by aerosol can be minimized, which is particularly important when measurements of stratospheric ozone take place in polluted areas. This type of AD-wavelengths pairs measurements has been commonly used in the network since the International Geophysical Year (IGY) (Dobson, 1957a, 1957b), when the manuals of Dobson spectrophotometers were written, describing the measurement technique, as well as test and calibration methods. These manuals (Evans, 2008; Komhyr, 1980) are still the basis ofthe measurements of the present network. Several continuous ozone time series started in the IGY. The measurements were collected by the secretary of IO3C and later by an archive, which is operated today as the World Ozone and Ultraviolet radiation Data Centre (WOUDC) (http://www.woudc.org/) by Environment and Climate Change Canada.
3 Stratospheric ozone in global atmospheric watch
In the early 1970s, the discussion about anthropogenic ozone depletion was raised, first discussing the emission of nitrogen oxides (NOx) from supersonic passenger aircrafts (Johnston, 1971) (a technology that turned out not to be successful), followed by the discovery that chlorine radicals could destroy stratospheric ozone (Molina and Rowland, 1974; Stolarski and Cicerone, 1974). Molina and Rowland were the first to realize that chlorine in the stratosphere might originate from the photolysis of chlorofluorocarbons (CFCs), which were increasingly used in the 1970s, e.g., as propellants in spray cans. However, chemical industry producing these compounds questioned the findings of Molina and Rowland, leading to an extended debate, in which Du Pont, the market leader of CFC, made a public advertisement in New York Times, 1975: “Should reputable evidence show that some fluorocarbons cause health hazard through depletion of the ozone layer, we are prepared to stop production of the offending compounds.”
In 1985, the Ozone Hole was discovered by Farman et al. (1985), and within a few years it was possible to demonstrate that it was caused by anthropogenic emissions of ozone-depleting substances (Solomon, 1999; Solomon et al., 1986). The Ozone Hole and the documentation of downward trends in stratospheric ozone (see below) can be viewed as “reputable evidence” of the impact of CFCs on the ozone layer, first theorized by Molina and Rowland in 1974.
Determination of significant ozone long-term trends from field measurements requires very high calibration stability of the network as stratospheric day-to-day variability in extra-tropics is large, amounting up to ±20%, while long-term ozone changes were estimated to be on the order of few percent per decade. The global Ozone Observing System (GO3S), which was later integrated in the Global Atmosphere Watch (GAW) program of the World Meteorological Organization, solved this problem in the following way: the primary Dobson instrument (Fig. 2) maintained by NOAA in Boulder is regularly absolutely calibrated by the Langley plot method during measurement campaigns taking place in the Mauna Loa Observatory in Hawaii, where conditions for such measurements are optimal (Komhyr et al., 1989). The stability of this instrument derived from these campaigns is excellent (Fig. 3), showing that the absolute drift since 1975 is less than ±0.5%. The calibration scale is subsequently transferred to the regional standard instruments and finally from the regional standard Dobson instruments to the station instruments by means of operating the instruments in parallel over a large range of solar angles (Fig. 2). The transfer of calibration from one instrument to another by this method introduces an uncertainty of ±1% for direct sun measurements, implying that the calibration stability of the network is expected to be around ±1%. Although this high calibration stability cannot be applied to individual Dobson records (Scarnato et al., 2010; Staehelin et al., 1998), the standard lamps are used to track their stability between calibrations. In case a drift is detected outside of the ±1% record, it is post-corrected after the calibration. Therefore, the regular application of instrument calibrations led to a strongly improved performance of the network (Fig. 4).

Scheme of the global Dobson calibration system, see text.
Personal communication Glen McConville, NOAA.

Results of the Langley plot calibration at Mauna Loa Observatory, Hawaii, and other locations demonstrating the excellent calibration stability of World Primary Dobson instrument maintained by NOAA, Boulder, Co (Mu is the relative slant path through ozone layer or airmass factor; Mu = 1/cos(ϑ), where ϑ is solar zenith angle (SZA)).
Evans et al., 2004 and personal communication Glen McConville, NOAA, Boulder, USA.

Improvement of the calibration of the Dobson network by regular intercomparison with Dobson standard instruments. The individual points show the deviation of the calibration of station instruments from the standard instruments prior to recalibration.
Provided by courtesy of Köhler et al., 2004, updated.
A similar calibration concept is used for the network of Brewer instruments (Brewer, 1973; Kerr et al., 1981), which are completely automated sun spectrophotometers commercially available since the middle of the 1980s using more modern technology (Fioletov et al., 2005). Ozone sondes and Umkehr profile data are additional measurements that belong to the GAW-ozone measurements.
In the second part of the 1980s, a debate about the reliability of the short TOMS satellite record took place (Heath, 1988). These TOMS data suggested a strong ozone downward trend also at mid-latitudes, contrasting with the results of ground-based Dobson instruments. These differences were evaluated in the International Ozone Trend Panel Report (IOTP, 1988). It turned out that the retrieval of TOMS as used in these early versions did not properly account for the degradation of the diffuser plate. The long-term trend analysis based on measurements by the ground-based Dobson instruments proved to be more reliable (for analysis of later retrieval algorithm of TOMS data, see, e.g., Stolarski et al., 1992).
However, IOTP disclosed some incoherence in the ground-based data. Satellite overpass data of TOMS were thus used as transfer standard in order to identify sudden breaks that occurred rather often in many ground-based Dobson series, most probably attributable to technical problems of station instruments including instruments’ calibration. Rumen Bojkov of WMO applied corrections to the ground-based measurements data (called “provisionally revised data”). Many of these records were subsequently homogenized by the station personnel. The ground-based measurements were analyzed in the PhD thesis of Neil Harris (Harris, 1989) showing for the first time significant negative (winter) trends in total ozone at northern mid-latitudes. These results were published in IOTP (1988) (Fig. 5). In this study a statistical multiple regression model was developed, allowing for determination of seasonality of long-term trends using explanatory variables to represent (natural) variability — the same type of model (ignoring proxy for nuclear bomb test) was subsequently used in all WMO/UNEP ozone assessments (starting with WMO/UNEP, 1989).

Ozone downward trends of northern mid-latitude Dobson stations from the IOTP, 1988.
4 Post Montreal Protocol measurements
The Montreal Protocol was signed on September 16, 1987 as the political agreement for a global reduction of the emission of ozone-depleting substances (ODS) linked to the Vienna Convention for the protection of the ozone layer (1985). The minimal requirements for the restrictions in emissions of ODS in the Montreal Protocol were initially weak, leading only to a slower growth of ODS concentrations in the stratosphere, but subsequent amendments of the Protocol strengthened the regulatory measures, leading to an effective removal of ODS from the stratosphere. Under the current regulations, stratospheric ODS concentrations are predicted to recover back to the 1980 levels around 2050 (WMO/UNEP, 2014). According to analyses published by Newman et al. (2009), stratospheric ozone would have been strongly damaged without the Montreal Protocol, potentially leading to a 67% reduction of the global ozone layer in 2065 as compared to 1980. In 1995, Paul J. Crutzen, Mario J. Molina, and F. Sherwood Rowland received the Nobel Prize of Chemistry for their outstanding contribution to atmospheric chemistry, particularly concerning the formation and decomposition of ozone.
Since the Montreal Protocol was implemented, the “turn-around” of downward ozone trends and thereafter the subsequent expected increase in stratospheric ozone levels (Fig. 6) are the highest priorities of the international and national organizations that are responsible for the monitoring of the ozone layer. Reliable ozone profile measurements are of great importance, particularly observations in the upper stratosphere, where increases in ozone at the mid-latitudes are viewed as one of the first signs of stratospheric ozone recovery (see, e.g., Steinbrecht et al. (2017) and references therein). Reliable long-term ground-based measurements are also becoming more and more important to evaluate composite satellite records.

World longest total ozone series (annual mean values) measured in Arosa (Switzerland) (Staehelin et al., 2018).
The evolution of stratospheric ozone is influenced by the decrease in ODS and the ongoing climate change. Climate change might accelerate the Brewer–Dobson circulation (Butchart, 2014), leading to higher column ozone at mid-latitudes and lower column ozone in the tropics. Current chemistry–climate models predict a return of total ozone at northern mid-latitudes to the 1980 levels by around 2030 (Dhomse et al., 2018), strongly depending on greenhouse emissions. Recently, Ball et al. (2018) developed a new method for merging satellite total ozone data and did not find evidence of an increase in the total ozone combined satellite records, averaged between 60°S and 60°N. On the contrary, they detected a continuous decline in the lower stratospheric ozone since 1998 between 60°S and 60°N latitudes. These results underline the importance to continue high-quality ozone measurements, keeping in mind that (i) the recovery in total ozone needs confirmation (Fig. 6), and (ii) downward trends reported by Ball et al. (2018) are not found by some CCM, requiring further investigation to identify whether dynamical or chemical mechanisms are responsible for the observed ozone evolution over mid-latitudes.
5 Key results of the NDACC network
The discovery of the Ozone Hole and the subsequent Montreal Protocol amendments prompted the set-up of other ground-based networks for the monitoring of ozone and related species. The Network for the Detection of Stratospheric Changes (NDSC) was established in 1991 (Kurylo, 1991). Based on the use of the most recent techniques for the measurements of atmospheric compounds and parameters in the stratosphere, it was built through the collaboration between national and international agencies.
Given the global aspect of stratospheric ozone depletion, the locations of NDSC stations were selected to provide a large latitudinal coverage, considering also the constraints of national funding. In 2005, NDSC extended its scope to the composition of the free troposphere and became the Network for the Detection of Atmospheric Composition Change (NDACC). At present, the NDACC's goals are:
- • establish long-term databases for detecting changes and trends in atmospheric composition, and understand their impacts on the mesosphere, stratosphere, and troposphere;
- • establish scientific links and feedbacks between changes in atmospheric composition, climate, and air quality;
- • validate atmospheric measurements from other platforms (i.e. satellites, aircraft and ground-based);
- • provide critical datasets to help fill gaps in satellite observations;
- • provide collaborative support to scientific field campaigns and to other chemistry and climate-observing networks;
- • provide validation and development support for atmospheric models.
NDACC includes more than 90 stations providing high-quality measurements of atmospheric composition at a global scale. The co-location of various instruments in the same station is favored to enable a better understanding of the causes of observed changes in the measured parameters. Fig. 7 shows the actual geographical coverage of the network. More information on the NDACC sites and archived data can be found on the NDACC website: http://www.ndsc.ncep.noaa.gov/.

Map of active NDACC measurement sites.
The network is organized in working groups based on the instrumental techniques selected within NDACC, e.g., lidar remote sensing, UV/Visible spectrometers, Fourier Transform InfraRed spectrometers (FTIR), microwave spectrometers, ozone sondes, Dobson and Brewer spectrophotometers, UV spectroradiometers. It is important to note that NDACC coordinates the worldwide measurements, but the instruments are operated and supported by national agencies and organizations. The NDACC also takes care of the collaboration and coordination between the stations, provides support and maintains online data base and fosters the preparation of and compliance with SOPs in order to gain the highest possible data quality. For a recent review about NDACC, we refer to the AMT/ACP/ESSD inter-journal special issue at https://www.atmos-chem-phys.net/special_issue400_819.html.
5.1 Data quality issues
Strict quality-control procedures have been implemented within the NDACC by the various instrument-working groups. They include comparisons of retrieval algorithms, as well as field intercomparison campaigns in order to verify the correct operation of the instruments at the various sites (see the NDACC website for a compilation of results of the various intercomparison campaigns). Such campaigns generally include a “blind” period, during which exchange and discussion of measurements are prohibited. They are supervised by a neutral referee, who is not involved in the campaign. The first formal inter-comparison of NO2 measurements by UV/Visible spectrophotometers was organized at Lauder, New Zealand, in 1992 (Hofmann et al., 1995). Similar intercomparison campaigns have been organized throughout the years by the microwave, FTIR, lidar and spectral UV working groups. For lidar instruments, generally not transportable, a mobile lidar system was developed by NASA for inter-comparison campaign purposes (McGee et al., 1991).
5.2 Tracking changes in ozone related species
Observations of hydrochloric acid (HCl) and chlorine nitrate (ClONO2) total columns by FTIR spectrophotometers are used to monitor the evolution of the stratospheric total inorganic chlorine (Cly), resulting partly from the breakdown of CFCs and their first range of substitutes, the hydrochlorofluorocarbons (HCFC). Thus, time series from NDACC stations spanning more than 30 years allow us to evaluate compliance to the Montreal protocol's regulation of halocarbons. These long-term records have provided evidence for the stabilization of the total inorganic chlorine from the end of the 1990s, following a rapid increase during the 1980s. Fig. 8 shows the NDACC time series from the Jungfraujoch station (Switzerland) measurements extending through 2016. It indicates that Cly has decreased slowly since it peaked in late 1996. Wiggles in the recent time series, with surprising increases in HCl of up to about 3%/year between 2007 and 2011 were shown to be caused by changes in atmospheric circulation at a global scale (Mahieu et al., 2014). Similarly, NDACC performs long-term measurements of the stratospheric bromine abundances (Hendrick et al., 2008).

Monthly mean total column time series of HCl (red circles) and ClONO2 (green triangles), monitored at the Jungfraujoch station (Swiss Alps, 46.5°N, 3580 m a.s.l.) in the framework of the NDACC network.
Courtesy: E. Mahieu et al., University of Liège, Belgium.
5.3 Tracking ozone profile trends
Outside the Polar Regions in winter and spring, the contribution of chlorine radicals (ClOx) to stratospheric ozone depletion is maximal in the upper stratosphere (WMO/UNEP, 1998). This region is thus expected to be one of the most sensitive regions to total chlorine decline following the Montreal Protocol. All measurement time series starting before 1990 show the stabilization of ozone levels after a decline in the earlier period and some sign of recovery in the most recent years.
Long-term measurements of ozone by Dobson Umkehr measurements, lidar, microwave, and FTIR spectrophotometers performed within NDACC and GAW have been used to evaluate the evolution of ozone in the upper stratosphere over the last decades (Harris et al., 2015; Hassler et al., 2014; Steinbrecht et al., 2017). Fig. 9, displaying the evolution of upper stratospheric ozone relative anomalies (near 2 hPa, ∼42 km), indicates an increase that started in the late 1990s. Anomalies are referenced to the 1998 to 2008 climatological annual cycle of each individual data set, and are averaged over the indicated zonal bands. The corresponding zonal averages from merged satellite records indicated in Fig. 9 (e.g., GOZCARDS — Global OZone Chemistry And Related trace gas Data records for the Stratosphere, https://www.gozcards.jpl.nasa.gov/info.php), SWOOSH (The Stratospheric Water and OzOne Satellite Homogenized; https://www.esrl.noaa.gov/csd/groups/csd8/swoosh/), SAGE + CCI + OMPS (http://www.esa-ozone-cci.org/) and two composites of SBUV observations (https://www.acd-ext.gsfc.nasa.gov/Data_services/merged/) are also included. The period 1998 to 2008 was chosen as the reference because ozone values were fairly constant over this period, and many instruments provide data for a substantial fraction of the period.
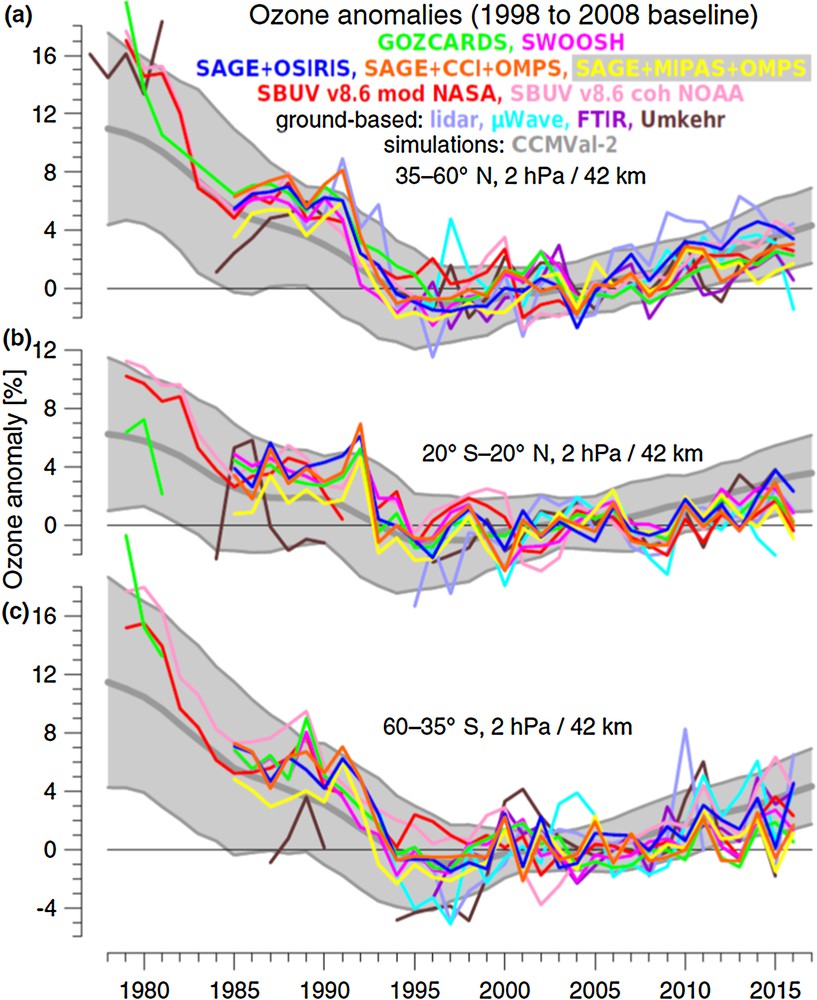
Annual mean ozone anomalies near 2 hPa or 42 km, as recorded by merged satellite data sets and ground-based stations (see text). Grey lines show the multimodel mean ozone anomalies from CCMVal-2 simulations (Eyring et al., 2010), with the grey shading giving the 2 σ standard deviation envelope.
Reproduced from Steinbrecht et al., 2017.
5.4 Validation of global satellite ozone profile measurements
Long-term NDACC observations of ozone vertical distribution are particularly useful to evaluate the precision and accuracy as well as possible drifts of satellite measurements records. Hubert et al. (2016) assessed long-term observation records of 14 limb and occultation satellite sounders by comparing them against observations of NDACC/GAW ozonesondes and stratospheric ozone lidars. Results (Fig. 10) showed that the best agreement between these measurements with lidar observations is obtained in the 20–40-km altitude range with biases smaller than ±5%, short-term variabilities less than 5–12% and drifts not exceeding ±5%/decade or even ±3%/decade for some records. The assessment of the drift of satellite instruments is particularly important for estimating global ozone trends after the peak of ODS in the stratosphere.

Overview of the drift (left column) and associated 2 σ uncertainty (right) of all satellite ozone records relative to the lidar (top row) and ozonesonde (bottom) NDACC/GAW networks. The dashed lines indicate instruments that ceased operations prior to 2006. The analysis is done in the native system of each satellite record. The decadal stability of most records remains within ±5% per decade in the middle and upper stratosphere (shaded area). POAM II and SAGE III drift results are not displayed in the lower left panel to avoid clutter.
Provided by courtesy of Hubert et al., 2016.
6 Ozone measurements in Antarctica
There are currently multiple ground-based stations (i.e. Dobson, Brewer, UV–VIS spectrophotometers, lidars and ozonesondes) regularly monitoring long-term ozone and the concentration changes of the compounds causing the Ozone Hole over Antarctica (see map in Fig. 11). Many stations contribute with near-real-time data to the NDACC database and to the WMO Antarctic ozone bulletins (see, e.g., http://www.wmo.int/pages/prog/arep/WMOAntarcticOzoneBulletins2016.html). The quality-assured ozonesonde, Dobson and Brewer data are regularly archived in the NDACC archives or in WOUDC. Long-term series such as Dobson measurements (stored in WOUDC including Halley Bay (1957–present) and Syowa (1961–present)), and NDACC database (Dobson data of the South Pole (1963–present) and Arrival Heights (1988–present)) were crucial to discover the Ozone Hole and to detect the anthropogenic ozone depletion in the 1980s (Chubachi and Kajiwara, 1986; Farman et al., 1985; Komhyr et al., 1986). The depletion in the ozone column appears during the austral spring after enhanced levels of chlorine and bromine compounds initiate ozone-depleting reactions. The reactions occur on the surface of polar stratosphere clouds that form in extremely cold temperature within the polar vortex. In order to understand and document the mechanism of ozone layer depletion, there is a need for vertical atmospheric profiling before, during, and after the depletion period (Fig. 12).

Map of Antarctic stations measuring ozone (Braathen, 2015).

Vertical profiles of ozone captured by ozonesonde measurements at the South Pole in 2015. Three stages of ozone depletion are provided: winter profile before sun activation of ozone destruction (blue), beginning of ozone destruction in September (green), and completely destroyed ozone between 15 and 18 km altitude in mid-October (red).
Five NDACC stations have been launching ozonesondes since the middle of the 1980s to collect vertical profiles of stratospheric ozone and temperatures in Antarctica, i.e. McMurdo (1986–2010), Dumont d’Urville (1991–present), South Pole (1986–present), Belgrano (1999–present), and Neumayer (1992–present) stations. These long-term records allowed researchers to understand the evolution and depth of the Ozone Hole in the past, prior to 2000, and to detect the beginning of ozone recovery in the most recent years (Solomon et al., 2016). Fig. 13 shows inter-annual variability in total, partial ozone column (between 12 and 20 km) and temperatures (20–24 km altitude) in 2016 (red) and 2017 (blue), as recorded by Dobson and ozonesonde instruments at the South Pole station. The slope in the ozone change during September indicates the presence of ozone-depleting substances in the stratosphere and defines the rate of seasonal ozone depletion (Hassler et al., 2011; Hofmann et al., 1997; Hofmann et al., 2009). The slope has been stable in the stratosphere since the 1990s, but is expected to decline in the future, thus indicating ozone recovery.

Ozone and stratospheric temperature data at the South Pole station. Total column ozone data from balloon soundings are shown as the larger symbols connected with a line (a). Dobson ozone data are shown as small size symbols (not connected) along the ozonesonde integrated ozone column record. The red line represents the data for 2017 and the blue line for the previous year (if available). The lines and shading follow a progression from bottom to top as the minimum (thin black line), the 10th percentile (light gray shading), 30th percentile (dark gray shading), median (thick black line), 70th percentile (dark gray shading), 90th percentile (light gray shading), and the maximum (thin black line) over the period shown in the lower left corner. Partial ozone column record between 12- and 20-km altitude collected in 2016 (blue dots) and 2017 (red dots) (b). Mean temperature between 20 and 24 km altitude (c). Polar stratospheric clouds form at −78 °C.
Near-real-time plots have been provided by courtesy of Global Monitoring Division at NOAA, Boulder (https://www.esrl.noaa.gov/gmd/dv/).
Fig. 13 also shows the range of the long-term ozone and temperature variability observed at NOAA South Pole Observatory since 1986. The grey envelope and the black line represent the mean and 2 σ standard deviation variability derived from the ozone-sonde record. Dobson ozone measurements with the standard solar technique are taken during the October–March period, when the sun is relatively high above the horizon. During polar winter (April through early September) Dobson ozone measurements are also possible during short periods when the full moon is used as the source of light. Continuous monitoring of ozone levels with the ozone-sonde technique started at the South Pole in 1986. Ozone observations from the earlier period (since 1957 and prior to 1980s) were taken by Dobson, ozone filter and ozonesonde techniques at Faraday, Vernadsky, Halley, Mirny, Hallet, South Pole, Byrd, and Base King Baudouin stations. These data provide information about the levels of stratospheric ozone in Antarctica prior to anthropogenic depletion that were detected by ground-based measurements in the 1980s.
Having this reference information of Antarctic ozone levels in the past is of importance for the international research communities who continuously monitor ozone levels to verify complete recovery of the ozone layer expected for 2060s (WMO/UNEP, 2014).
7 Conclusions
The pioneering work by Fabry and Buisson in Marseille (France) at the beginning of the 20th century and later by Dobson in the UK created robust, sensitive instrumentation and developed early methods for the ground-based monitoring of the stratospheric ozone layer. The early observations made with these instruments helped establish seasonal ozone variability at various latitude ranges and connect total column ozone changes with Brewer–Dobson circulation in the stratosphere. The long-term ozone monitoring began in the 1920s at Arosa (Switzerland), at first with emphasis on human health and then to understand climatological changes in ozone (Staehelin et al., 2018). The WMO International Geophysical Year 1957 called for expansion of ozone observations, which later resulted in the establishment of the WMO global ozone network. The benefit of the global ozone observations was recognized in the 1980s as an important tracer to monitor the effects of increasing anthropogenic activities and their global impact on UV levels, human health, ecosystem, and Earth climate. The observations of the Dobson network played a key role in the discovery of the Ozone Hole in the 1980s. The historical ozone observations prior to 1980s are used as a reference for the assessment of ozone recovery linked to the enforcement of the Montreal Protocol.
This paper also discusses the most important results of the long-term ozone observations performed under the GAW and NDACC networks. In order to detect a few percent per decade change in stratospheric ozone records, the monitoring networks are required to demonstrate long-term stability and provide high-precision measurements. The approach taken by the WMO GAW program enabled the calibration stability of the network to attain a range of ±1%. Dobson spectrophotometers, together with other instruments measuring total ozone columns, provided reference for the evaluation of satellite records and assistance in creating combined global records. NDACC has also implemented strict data protocols for its observations in order to ensure the high quality and consistency of the data at a global scale and on the long term. It is important to mention the complementary roles of ground-based networks and satellite instruments going back to the important results of the International Ozone Trend Panel Report (1988), which showed, for the first time, ozone decreases at mid-latitudes during winter time.
Since the early 1990s, the ground-based monitoring activities under the WMO GAW and NDACC programs have provided support for the amendments of the Montreal Protocol. Co-location of multiple instruments at the NDACC and GAW stations allows for collection of complementary information needed for the attribution of changes in observed ozone records (i.e. CFC's, NOx, etc.). The importance of continuous observations in the stratosphere with multiple observing systems warrants the observational redundancy and thus assures their stability. The archival of data in the WOUDC and NDACC data hosting facilities preserves ozone records for the community and provides tools for sharing information with users, including near real-time provisions of data for air-quality and climate model predictions.
High-quality ground-based measurements are indispensable to evaluate ozone variabilities and long-term trends, assess chemistry climate models and check the long-term stability of satellite data, including more recently the merged satellite time-series developed for the detection of ozone recovery at global scale, in a changing climate. The continuation of international and national support for acquiring and archiving ground-based records is of great importance for atmospheric research. It is mandatory for monitoring the compliance of countries to the Montreal Protocol and associated amendments, as well as for tracking and reporting on the observed rates of ozone recovery. Some current geoengineering projects, designed as solar radiation management, are threatening the recovery of the ozone layer in the context of still high levels of halogen compounds in the stratosphere (Nowack et al., 2016; Tilmes et al., 2008). Therefore, multiple atmospheric composition measurements from the NDACC and GAW networks can guide Montreal Protocol parties in the assessment of the potential impacts of geoengineering on the health of the stratospheric ozone layer.
Acknowledgments
The authors want to acknowledge the invaluable contributions of all national and international agencies and authorities for their support of ground-based monitoring of the atmospheric composition and of ozone in particular (for example NASA, BAS (British Antarctic Survey), and ESA). We furthermore acknowledge the contributions of the European Commission (for example, the Arctic campaigns EASOE, SESAME… and EU projects related to atmospheric monitoring such as REVUE and CANDIDOZ). We also thank WMO/GAW and NDACC for support in archiving data and making sure that quality assurance is implemented through the responsible working groups as well as all the researchers and operators who have devoted part of their life to atmospheric research.