1. The services the biosphere provides to humanity are deteriorating as it loses its biodiversity
For this article, I wanted to use the term biosphere rather than biodiversity or ecosphere for the following reasons: the term biosphere, first introduced by geologist Eduard Suess in 1885 [Suess 1885], represents all living organisms and the environments in which they live. It therefore includes both the notion of biodiversity, which represents the diversity of living organisms, including its taxonomic diversity and its genetic diversity; and that of an ecosystem, which represents a community of living beings that interact with each other and with their environment. The terms ecosystem and biodiversity appeared decades later (1935 and 1986 respectively) and gradually supplanted the term biosphere, probably for convenience in public debates, allowing a more precise discussion of the various environmental issues humanity is facing (ecosystem services, endangered species, genetic resources, etc.). The equivalent of the IPCC (Intergovernmental Panel on Climate Change) for the biosphere is the Intergovernmental Science-Policy Platform on Biodiversity and Ecosystem Services (IPBES). Most of the examples I will cite in this article will concern the terrestrial environment. For a more specific overview of the marine environment see for example Gattuso et al. [2018], Hoegh-Guldberg and Bruno [2010].
The biosphere provides a very large number of services to humanity, which could be described as vital, as its health, food and well-being depend on it. There are generally four main types of services provided to humans by the biosphere: provisioning services (food, water, raw material, energy), regulating services (air quality, soil fertility, flood and epidemic control, etc.), cultural services (cultural identities, aesthetic and spiritual values, source of artistic and scientific inspiration), and supporting services which are the basis of all ecosystems and their services (favourable habitats for animal and plant species participating in ecosystem services and preservation of their diversity).
Most of the services provided to humans by the biosphere have only deteriorated over the last fifty years, and in particular the services of pollination, genetic resources, disease vector regulation, and soil fertility [Díaz et al. 2019]. The deterioration of these services is largely due to the fact that the biosphere loses its biodiversity, in terms of the number of species and ecosystems, and genetic diversity [Díaz et al. 2019; Oliver et al. 2015a]. The rate of species extinction is currently at least ten times higher than it has been in the last ten million years and it is only increasing [Díaz et al. 2019]. This loss of diversity affects species both wild and domesticated by humans [Díaz et al. 2019; FAO Commission on Genetic Resources for Food and Agriculture 2019]. This rapid decline in biodiversity means that most of the goals of international agreements on the environment and human well-being (Aichi Targets of the Convention on Biodiversity, United Nations Sustainable Development Goals 2030) will not be achieved on the basis of current trajectories [Díaz et al. 2019]. This decline will also undermine the goals specified in the Paris Agreement adopted at COP21 (21st Conference of the Parties to the United Nations Framework Convention on Climate Change).
2. Climate change is currently the third leading cause of biodiversity loss
The current primary causes of biodiversity loss are the destruction of natural habitats by human activities, direct exploitation for food, health and the creation of material goods, and climate change, which ranks third ahead of pollution and biological invasions [Díaz et al. 2019]. Climate change is currently exacerbating the effects of other human pressures on the biosphere and has become the major cause of changes in the biosphere in recent decades [Field et al. 2014]. These changes are mainly a modification of the development cycles of living beings, which has major consequences on their growth, survival and reproduction, a modification of the geographical ranges of species, and a modification of the exchanges of water, gas and energy fluxes between the biosphere and the atmosphere [Pecl et al. 2017].
Climate change is changing the course of the annual development cycle of both plant and animal species, including so-called "poikilotherms" animals that do not regulate their body temperature (for example reptiles, amphibians, fish, insects, crustaceans, molluscs) [Cleland et al. 2007; Menzel 2003; Parmesan and Yohe 2003]. The dates of appearance, during the year, of the various events that punctuate the annual development cycle of these species, such as the appearance of new leaves or flowers in plants, the hatching of eggs, etc., are very closely dependent on the weather conditions that prevailed several weeks or even months before the appearance of these events [Bronson 2009; Chuine and Régnière 2017]. Global warming advanced the resumption of activity of living beings in spring by two to three days per decade, and delayed the end of their activity in autumn by one to two days per decade (Figure 1) (Menzel et al. 2006; Parmesan and Yohe 2003; Walther et al. 2005). However, while observed trends have been linear so far, recent studies show that since the 1990s, earliness trends in spring have slowed in plants [Fu et al. 2015]. This slowing down of the earliness trends of the resumption of activity of plants in spring is thought to be due to winter warming [Asse et al. 2018], as predicted by the models [Chuine et al. 2016; Morin et al. 2009; Vitasse et al. 2011] and experimental results [Morin et al. 2010]. Indeed, perennial plants need a certain number of cold days during the winter to stop the dormancy of their buds and to flower (floral initiation) [Anderson et al. 2010; Campoy et al. 2011]. This winter dormancy, which corresponds to a state of physiological inactivity regulated by internal factors, is an adaptation of extratropical plants to survive the bad season (winter in our latitudes) whose temperature conditions are not compatible with cellular activity. It is thanks to this adaptation in particular that the plants can withstand frost during this period. If the consequences of this lack of winter cold are for the moment a slowing down of spring earliness, the models predict that this lack of cold will cause in a few years a delay in the resumption of activity of the vegetation then in a few decades an impossibility to resume this activity normally [Chuine et al. 2016].

Illustration of the effect of climate change on the development cycles of living beings. Blue: winter rest period, green: period of activity and growth; orange: period of preparation for winter rest. During the winter resting period, most plants and tree buds are dormant, many animals are in diapause, wintering or hibernation: physiological activity is reduced to a minimum. Activity resumes in spring, and earlier than before (15 to 30 days depending on the species since the 1950s). The end of the activity period that allows preparation for the winter rest period comes in autumn and a little later than before (8 to 15 days depending on the species since the 1950s). These shifts in development cycles during the year resulted in damage to vegetation, particularly fruit crops, which are linked to late frosts, as young leaves emerge from the buds before the last spring frosts. They have also caused an increase in water scarcity during the summer as vegetation draws water from the soil earlier and longer than before. Finally, the cycle of each species is modified in a certain way and differently from other species, so that the cycles that were synchronised, as is often the case in trophic chains, are no longer synchronised, and many species are thus deprived of their main food resource.
These changes in the annual development cycle of living beings have many cascading consequences, some positive but many negative. In perennial plants, these changes are reflected in a lengthening of the growth period [Menzel and Fabian 1999], partly responsible for the increase in forest productivity until the early 2000s [Delpierre et al. 2009; Myneni et al. 1997; Piao et al. 2007]. On the other hand, in annual plants, the annual growth cycle is shortened because development is accelerated. This shortening of the annual cycle results in a decrease in the amount of flowers and seeds produced, even when water supply conditions are optimal, as this generally depends on the growth that may have occurred prior to flowering [Asseng et al. 2011; Ferris et al. 1998; Wardlaw and Wrigley 1994; Wheeler et al. 1996]. Changes in the annual developmental cycle of living organisms also have other consequences such as an increase in late frost damage as vegetation begins to grow before the arrival of the last spring frost (Figure 1) [Lenz et al. 2013; Vitasse et al. 2018], which in recent years has led to significant crop losses for wine and fruit growers. The lengthening period of activity also results in an increase in water scarcity, as vegetation depletes soil water supplies faster, as it begins to draw water earlier and for longer (for perennial species, including trees) (Figure 1) (Carnicer et al. 2011; Jump et al. 2017). This phenomenon occurs in some areas already facing increased and repeated summer droughts due to decreased precipitation and increased evapotranspiration due to increased temperature [IPCC 2013; Sheffield et al. 2012].
These changes in the development cycles of living organisms also impact interactions between species, in particular within trophic chains in which the prey-predator, host-parasite, plant-pollinator or even plant-herbivore relationships are determined by the stage of development of each of the interacting species (see for review Renner and Zohner, 2018). For example, the caterpillar stage of a butterfly species that feeds exclusively on young leaves must be perfectly synchronous with the young leaf stage for the survival of this butterfly species (Figure 1). However, the development cycles are not modified in the same way in the different species because they are not equally sensitive to temperature (Figure 2). Cycles are out of sync and food chains are breaking [Renner and Zohner 2018]. Consequently, beyond a modification of the trophic interaction networks [Gilman et al. 2010; Walther 2010], these desynchronisations of cycles between species will also cause species extinction [Cahill et al. 2013]. It is estimated that on average out of ten trophic chains, six species could disappear as a result of this desynchronisation [Koh et al. 2004].

Variation in species performance with temperature. The performance here represents both that of a physiological process, as well as the growth, survival or fertility of a species. The performance curves can take various forms but all show a thermal optimum, and decrease sharply when the temperature is too low or too high. The thermal optimum is generally variable between species, between individuals of the same species and also between physiological processes. Temperature response curves can generally vary to some extent in the very short term due to acclimation processes within the same genotype/individual, and in the longer term due to genetic adaptation in a species/population. The living beings can tolerate leaving their optimal thermal zone but for a time all the more limited as they are far from the optimum. Adapted from Figure TS 3.A of the [IPCC 2014] WG 2 report.
The second major impact of climate change on the biosphere, which is partly a consequence of the first, is species range shift [Parmesan and Yohe 2003; Walther et al. 2002]. The species move up to the mountain tops [Lenoir et al. 2008; Morueta-holme et al. 2015], and towards the poles [Beaugrand et al. 2003; Brommer et al. 2012; Hickling et al. 2006], such as the processionary caterpillar that moves up towards the North of France at an average speed of 5.5 km/year and towards summits at a speed of 5 m/year [Battisti et al. 2005]. These species movements occur at variable rates depending on their migration capacity. Sessile organisms such as plants are able to migrate at a maximum speed ranging from a few meters to thirty kilometers per decade, depending on the characteristics of their seeds which determine their dispersing agents (gravity, wind, water, animals). In comparison, large mammals, winged insects and pelagic marine organisms can migrate hundreds or even thousands of kilometres per decade. These range shifts also result in local extinctions of species in the warm margins of the ranges of many species [Parmesan and Yohe 2003; Walther 2003]. The species that live in areas limited to polar regions and subalpine levels are particularly vulnerable because their habitat is inexorably shrinking [Callaghan et al. 2004; Engler et al. 2011], as are endemic species that have very restricted ranges. [Dirnböck 2011; Malcolm et al. 2006].
One of the fundamental reasons for all these effects of climate change on living organisms is that their physiological activity can only take place within a fairly narrow temperature range, of only a few tens of degrees (Figure 2). The performance of living organisms is zero when the temperature becomes too low or too high and they are optimal at an intermediate temperature level. Although it is not yet clear why the effectiveness of biological processes at the cell, organ or organism level varies so much with temperature, the effect of temperature on molecular structures probably plays an important role [Schulte 2015]. For example, regarding the development of plants in extratropical environments, we know that most of them need cold temperatures to break seed and bud dormancy, and to flower, indicating that the bad winter season has passed, and that growth can resume [Anderson et al. 2010; Campoy et al. 2011]. We also know that once this dormancy ends, the warmer the weather, the faster the cell growth is, until reaching a thermal optimum that can vary between 20 and 30 °C depending on the species [Anderson et al. 2010]. Beyond this optimum, growth performance decreases even under conditions of optimal hydration until reaching a lethal thermal threshold beyond which proteins and nucleic acids are denatured and cellular membranes disorganised, causing irreparable cellular damage and death of the organism. Poikilothermic animals (which do not regulate their body temperature) also depend on external conditions for their development. The rates of embryonic and larval development depend on outdoor temperature in a relationship presenting an optimum [Cossins and Bowler 1987; Gillooly et al. 2002] (Figure 2). Each temperature-dependent biological process has its own thermal optimum that varies between species and between individuals of the same species. The aggregation of all these processes allowing organisms to live and reproduce results in a relationship of survival and fecundity rates to temperature which also has a thermal optimum and which varies between species and between individuals.
We are currently very far from knowing what are the thermal optima and lethal thermal thresholds of all species. Nevertheless, it must be noted that these lethal thermal thresholds are increasingly being reached. For example, it appears that the functioning of vegetation is being severely compromised in many parts of the world due to recurring heat waves and droughts that regularly break records [Field et al. 2014]. When conditions become too dry and too hot, vegetation will initially change from a carbon sink to a carbon source because it will breathe more than it photosynthesises. [Bonan 2008; Chapin et al. 2008]. And if these conditions become too frequent or too intense, it will then wither and die [Carnicer et al. 2011; Jump et al. 2017]. Water availability is just as crucial to cell function, if not more so, than temperature conditions. Cell growth requires in plants a certain level of cell hydration and during a dry episode, it is one of the biological processes that will stop first [Cosgrove 1986; Lempereur et al. 2016]. However, the availability of water is also greatly affected by climate change, on the one hand, because the precipitation regime has changed in many regions (a clear decrease in the South of France for example); and on the other hand, because the increase in temperature increases soil evaporation and vegetation perspiration. Beyond water availability issues, the June 2019 heat wave revealed that even under no water scarcity conditions, high temperatures could cause significant damage to vegetation. This heat wave has caused unprecedented damage to the foliage of many species, including Mediterranean species particularly resistant to high heat and drought such as the holm oak (Figure 3). Although the causes of this damage have not yet been analysed, the hypothesis put forward is that they are due to the exceptional earliness of the heat wave for which the plants were not prepared, many of which have not yet completed the installation of the various protective structures of their leaves, such as the cuticle.
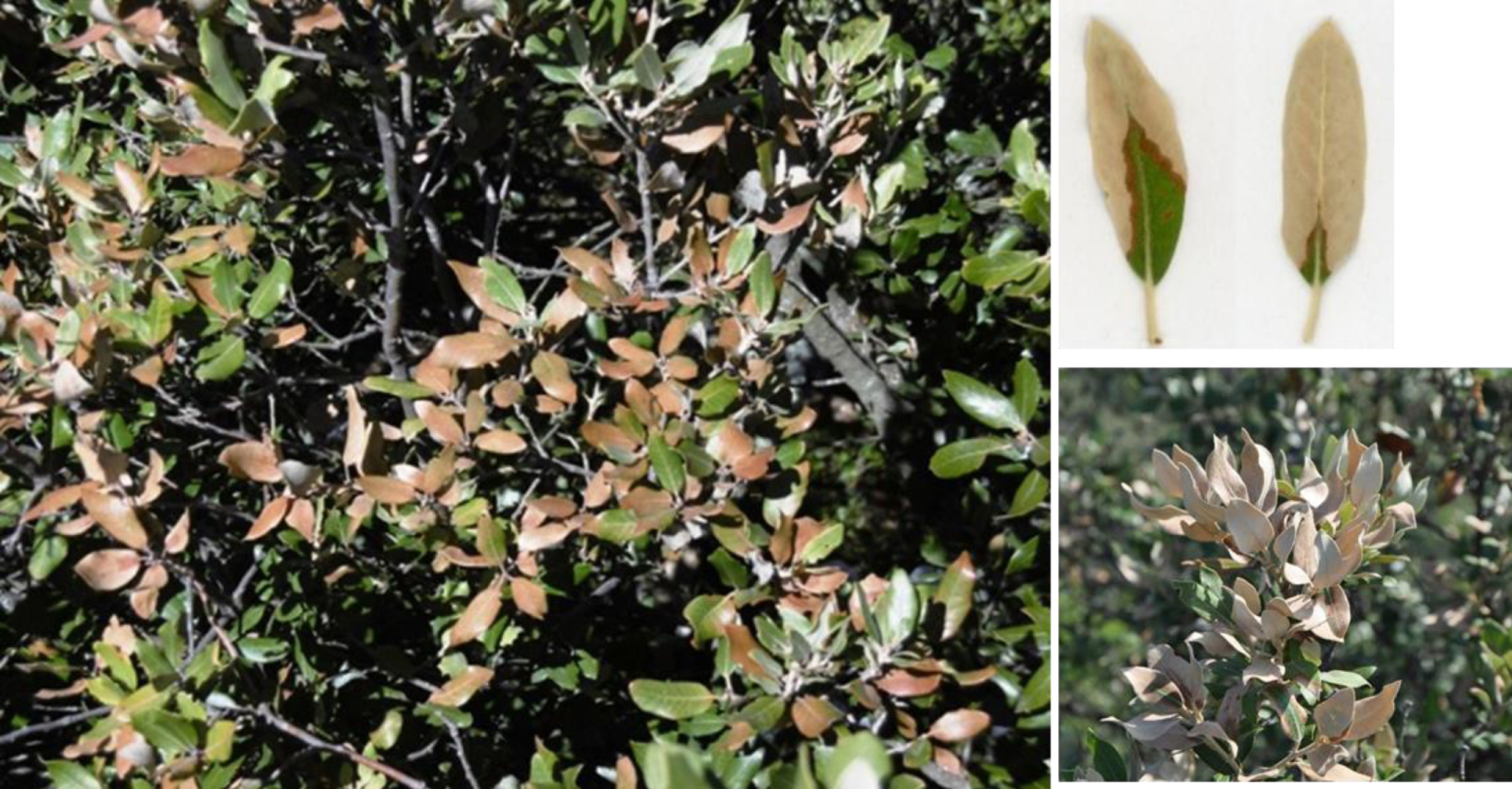
Photographs of holm oak leaves on the day after the heat wave of 29 June 2019 at the CNRS experimental site in Puéchabon (Hérault). The leaves turned white in a few hours before turning brown after a few days. The holm oak is a Mediterranean species adapted to heat waves and droughts. The damage caused by the heat wave of June 2019 is unprecedented and probably due to the exceptional earliness of the heat wave which arrived before the leaves had completed their development and in particular the building of protective structures such as the cuticle. Photos: Jean-Marc Limousin and Jean-Marc Ourcival, CNRS.
3. The effects of climate change on the biosphere have consequences for human well-being and activities, ecosystem services, and the climate itself
Beyond the consequences for the species themselves, the effects of climate change on the biosphere also have consequences for ecosystem services, human well-being and the climate itself.
The biosphere is affected by changes in the atmosphere, but the atmosphere is also impacted by the biosphere in many ways. Terrestrial vegetation is an important component of the climate system [Chapin et al. 2008; Marland et al. 2003]. It exchanges energy fluxes with the atmosphere, water and gas as well, mainly carbon dioxide and oxygen [Bonan 2008; Chapin et al. 2008]. These fluxes involve many physical, biochemical and physiological processes with complex regulatory patterns and feedback loops. Any change in vegetation or its functioning therefore leads to changes in these fluxes which affect surface temperature, air humidity, albedo, but also carbon stocks [Bonan 2008; Chapin et al. 2008]. For example, the increase in the abundance of woody vegetation in the Arctic tundra due to climate change decreases the surface albedo, and, as a result, the surface energy budget and ultimately the surface temperature, thus amplifying the already more pronounced warming at high latitudes [Chapin 2005]. This amplification effect adds to that of the reduction of the snow surface at high latitudes, which also reduces the surface albedo.
Warming and increasing atmospheric concentrations of carbon dioxide cause an increase in photosynthetic activity of plants that consumes carbon dioxide and produce oxygen. But warming also increases the respiration of plants, which therefore consume more oxygen [Grace and Rayment 2000; Ryan 1991]. The net carbon balance can therefore vary greatly from region to region depending on climatic conditions. It is positive in regions that still receive sufficient precipitation, but it is becoming increasingly negative in regions subject to increasingly long and intense droughts [Allard et al. 2005]. This is due to the fact that most plants can no longer photosynthesise when they lack water. Indeed, the plant absorbs carbon dioxide from the air by opening structures called stomata, generally arranged on the lower face of the leaves, but when open, the stomata also release oxygen (produced by photosynthesis) and water. These complex interactions between climatic conditions and vegetation functioning are extremely important to consider in our assessment of the role of vegetation, and in particular forests, in climate change mitigation [Richardson et al. 2010; Wood et al. 2012]. Consequently, the effects of climate change on the functioning of vegetation have both positive and negative effects on the climate itself, and it is necessary to have a comprehensive understanding of the many interactions involved to implement nature-based climate change mitigation solutions.
Climate change is profoundly changing one of the most essential ecosystem services for humans, which is the supply service (food, raw materials, drinking water and medicines). This effect occurs through range shifts of species, in particular marine species (fisheries resources); but also through the performance of species, in particular those exploited by humans, in terms of growth and reproduction, i.e. productivity for humans. The impact of climate change on field crops has so far been rather negative with declining yields, especially for wheat, rice and maize, which are the three largest crops in the world (http://www.fao.org/) [Field et al. 2014; Lobell et al. 2011; Schlenker and Roberts 2009]. These decreases in efficiency are due in part to the acceleration and shortening of the annual development cycle mentioned above. In this specific case of cultivated plants (but this also applies to domesticated animals), the loss of genetic diversity due to the reduction in the number of cultivated varieties, to keep only the most productive and the most attractive for marketing [FAO Commission on Genetic Resources for Food and Agriculture 2019; Wolff 2004], now hinders the selection of varieties better adapted to new climatic conditions [Wolkovich et al. 2018] and alter the ability of agroecosystems to withstand climatic hazards [Forest et al. 2015] and natural hazards such as pests and pathogens [Ekroth et al. 2019; White et al. 2020]. Indeed, taxonomic diversity and genetic diversity generally make plant and animal communities more resilient to hazards. [Oliver et al. 2015b]. This resilience is generally the result of redundancy and complementarity between different species, varieties, and genotypes, in the exploitation of resources (water, light, nutrients), and in the capacities of defense against pests and pathogens, and resistance to climatic hazards [Allan et al. 2011; Downing et al. 2014]. Taxonomic diversity and genetic diversity can also slow down the genetic adaptation of pests and pathogens to their hosts in the long term [White et al. 2020]. The use of positive interactions between species, varieties/breeds and genotypes is one of the fundamental principles of agroecology.
The effects of redistribution of species on the Earth's surface, resulting from climate change, on ecosystem functioning and community recomposition are less well documented and understood. Nevertheless, we know that this redistribution of species disrupts trophic chains in the same way as the modification of the annual development cycles mentioned above. For example, the recent arrival of the king crab, a formidable predator of echinoderms and molluscs, on the Antarctic seabed, which was previously inaccessible to crustaceans because the water temperature was too low, has profoundly altered the balance of this ecosystem, which has probably remained unchanged for millions of years [Aronson et al. 2015].
Finally, species range shifts due to climate change can also affect human well-being and health. For example, climate change is causing a range shift of many pathogen vectors, such as the anopheles mosquito, whose range is increasing as a result of climate change. As this species is a vector of malaria, the population exposed to malaria is increasing [Siraj et al. 2014].
4. Climate change is likely to become the leading cause of biodiversity loss and the means of adaptation are limited
Model projections predict that the negative effects of climate change on biodiversity will increase, in some cases exponentially [Bellard et al. 2012; Díaz et al. 2019]. Although extinction events will mostly be local (disappearance of a species from a region), the total extinction rate will also increase [Bellard et al. 2012; Thomas et al. 2004]. Even for global warming limited to 2°C, the models predict that the range of the majority of terrestrial species will decrease significantly, and that 5% of species will be at risk of total extinction, this number would increase to 16% with a warming of 4°C [Díaz et al. 2019]. Models also predict considerable changes in the functioning of ecosystems and their nature, even for a warming of 3°C in boreal regions and of 4°C in temperate regions [Heyder et al. 2011]. They also predict a sharp increase in forest decline in the temperate-boreal zone [Heyder et al. 2011]. Finally, they predict that marine environments will be as affected as terrestrial environments with an increase in the extinction rates of species in subpolar, tropical, and semi-enclosed seas, and a 60% turnover of local biodiversity that will affect the services provided to humans by marine ecosystems [Cheung et al. 2009]. Under these conditions, the capacity of protected areas to conserve endangered species will be greatly affected [Díaz et al. 2019; Monzón et al. 2011].
However, most projections do not take into account the adaptive capacity of species to climate change, as very few models are currently capable of doing so. The ways in which species adapt to climate change are essentially threefold: migration, phenotypic plasticity and genetic adaptation [Aitken et al. 2008; Davis and Shaw 2001; Lavorel et al. 2017]. Species can escape climate change by moving to territories whose climate remains or becomes favourable to their survival and reproduction, and thus escape total extinction (Figure 4). But if we compare the velocity of climate change to the velocity of species, we realise that many species cannot migrate as fast as the climate, especially plants (Figure 4). For example, the velocity of climate change under the RCP 8.5 scenario is greater than seventy kilometers per decade whereas the maximum known velocities of migration in plants are of the order of thirty kilometers per decade, when average velocities are one to two kilometers per decade [Field et al. 2014]. Thus, without assisted migration of individuals or propagules (e.g. seeds) by humans, many species will be at risk of total extinction before they have been able to escape from areas that will no longer ensure their survival. However, human-assisted migration has a significant cost and can only be implemented for a very limited number of species. Apart from conservation programmes for endangered species, assisted migration is currently being tested in forestry on species of economic interest [Aitken and Bemmels 2016].
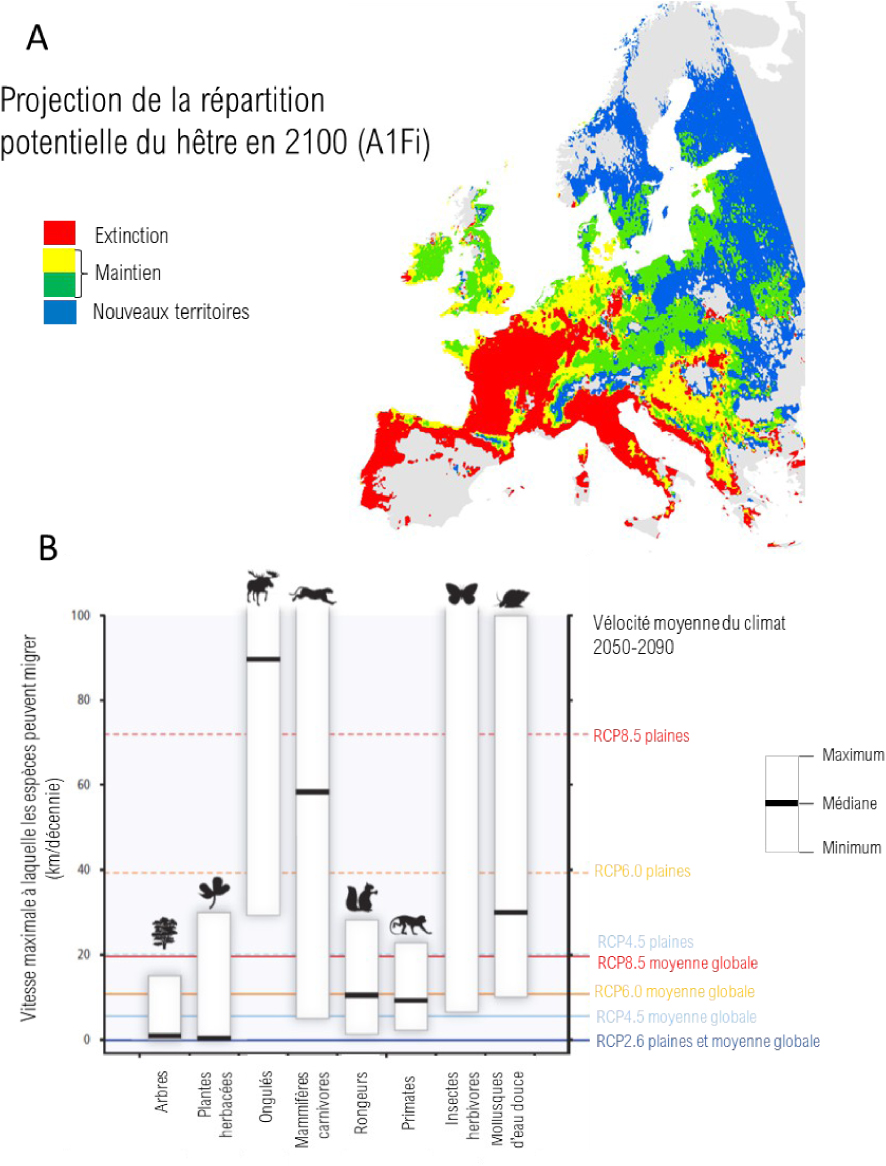
Impacts of climate change on species ranges. A. Projection of the potential range of beech in 2100 based on the A1Fi climate scenario and the PHENOFIT range model. Red: area where climatic conditions will no longer ensure the survival of the species, yellow and green: area where climatic conditions allow the survival of the species (green: more favourable conditions than before; yellow: less favorable conditions than before), blue: area where the climate allows the survival of the species and from which species was previously absent. Illustration adapted from Saltré et al. [2015]. B. Maximum speed at which species can migrate and climate velocity under different scenarios. Figure adapted from Figure SPM5 of the IPCC Working Group 2 report [Field et al. 2014].
Another way in which species adapt to climate change is through so-called phenotypic plasticity, where this is adaptive [Merilä and Hendry 2014]. Adaptive phenotypic plasticity is the ability to maintain a certain level of performance when conditions change, thanks to a modification of gene expression (Figure 3). For example, the growth rate of buds in the spring changes with temperature, and sprouting comes ever earlier as springs get warmer. But this plasticity is not always adaptive. Using models capable of describing the plasticity of the date of budburst, as well as the relationship between the date of budburst and tree performance, Duputié et al. [2015] showed that under future climatic conditions, the plasticity of the date of budburst will be very mostly advantageous in oak and will help this species to maintain itself to some extent under new climatic conditions, whereas it will be disadvantageous in Scots pine which risks disappearing from a large part of Western Europe because plasticity will have reached its limits in this species.
Finally, the ultimate adaptation to climate change is genetic evolution under the effect of natural selection brought about by climate change itself. Climate change is putting very strong selection pressure on living beings. Evidence of this is the existence of local genetic adaptations in wide-ranging organisms and high gene flow (which instead tend to genetically homogenise populations) [Alberto et al. 2013]. There is growing scientific evidence of genetic evolution under climate change [Merilä and Hendry 2014]. One of the easiest examples to understand is the change in daylength inducing winter diapause (a temporary state of greatly reduced physiological and metabolic activity) in the mosquito. In five years, the daylength inducing diapause in this mosquito has decreased by one hour in response to climate change, allowing the mosquito to continue its activity longer in autumn than before [Bradshaw and Holzapfel 2008]. This genetic change in mosquito sensitivity to the length of daylight to enter diapause is explained by the fact that warmer temperatures in autumn allow the mosquito to continue its activity and reproduction, and therefore it becomes advantageous to enter diapause later. Consequently, genes present in mosquito populations that induced diapause later than other genes present by responding to a shorter duration of the day were selected and propagated in the populations. But the mosquito has an extremely short generation time. It is able to produce several generations a year. Natural selection is therefore very effective in this species because the selection operates several times a year. Natural selection will also be more effective if genetic diversity in populations is high and population sizes are large. But not all species meet these conditions. Many species have very long generation times, such as many large mammals and trees. Furthermore, genetic diversity is often low in rare species [Gitzendanner and Soltis 2000] and has tended to decrease overall in human domesticated species [FAO Commission on Genetic Resources for Food and Agriculture 2019] [Wolff 2004] and species whose habitat area has been greatly reduced by human activities [Allentoft and Brien 2010]. Our ability to predict how quickly species will successfully adapt genetically to climate change is currently very weak as we are still far from understanding the genetic basis underlying the evolutionary responses to climate change (but see [Gauzere et al. 2020]). However, humans have two means at their disposal to accelerate natural selection in natural populations: to increase genetic diversity in populations by carrying out assisted gene flow, and to increase the areas of species' habitats in order to increase population sizes, for example by restoring degraded habitats or by increasing the areas of protected areas. These interventions, such as assisted migration, have significant costs. Nevertheless, the second lever of action, i.e. to increase the areas of the species' habitats, is already part of certain recommendations relating to the Sustainable Development Goals that France has ratified, and it will undoubtedly be the most efficient and the most sustainable.
5. Conclusion
Climate change profoundly affects the biosphere in many ways, as climatic conditions determine the performance of living organisms, their ability to reproduce and survive. These changes in the performance of living organisms consequently alter the functioning of ecosystems, the range of species, and biodiversity. Climate change is currently the third leading cause of biodiversity loss and risks becoming the first in the short term. The means of adaptation to climate change are limited for many species. As a result, beyond the necessary drastic reduction of greenhouse gas emissions to limit global warming, it is also necessary to take strong action on other causes of biodiversity loss and to implement nature-based solutions. Nature-based solutions are actions aimed at protecting, sustainably managing and restoring natural or modified ecosystems to directly address societal challenges in an effective and adaptive manner, while ensuring human well-being and producing benefits for biodiversity [IUCN 2018]. Only these solutions will restore and maintain the ecological and evolutionary processes that will enable species to cope effectively and sustainably with climate change. In addition, measures to mitigate climate change and protect nature can be synergistic (e.g. halting deforestation, reforestation in a reasoned manner, improving soil health, agricultural practices, etc.).