[La différenciation des planètes telluriques vue par la mécanique des fluides]
The basic structure of the terrestrial planets—an iron-rich metallic core surrounded by a silicate mantle—was established during their accretion, when widespread melting allowed the metal and silicate phases to separate. The transfer of chemical elements and heat between the metal and silicate that occurred during this period is critical for the composition and initial temperature of the core and mantle, and has important implications for the long-term evolution and dynamics of the planets. After having summarised the main observational constraints on core-mantle differentiation, the article follows the sequence of processes that led to the formation of planetary cores, focusing on the contributions of laboratory fluid dynamics experiments to our understanding of these processes, and discussing the relevance and limitations of this approach to this problem. We first focus on the dynamics of planetary impacts, using laboratory experiments to illustrate and quantify the impact and cratering processes and the resulting metal phase dispersion. We then consider the two-phase flow that follows an impact, when a molten impactor core falls by buoyancy in a magma ocean. The model of miscible turbulent thermal, which we argue is a good reference model for the post-impact flow, is presented. We then discuss how additional factors—immiscibility and fragmentation, inertia inherited from the impact, Coriolis force, sedimentation—affect the predictions of this model, and discuss the extent of chemical equilibration. Finally, a last part of the article is devoted to the migration of the metal phase through a solid part of the mantle.
La structure des planètes terrestres — un noyau métallique riche en fer entouré d’un manteau silicaté — a été établie pendant leur accrétion, lorsque la fusion généralisée a permis la séparation des phases métalliques et silicatées. Le transfert d’éléments chimiques et de chaleur entre métal et silicates qui s’est produit pendant cette période est déterminant pour la composition et la température initiale du noyau et du manteau, et a des implications importantes pour l’évolution et la dynamique à long terme des planètes. Après avoir résumé les principales contraintes observationnelles sur la différenciation noyau-manteau, l’article suit la séquence des processus qui ont conduit à la formation des noyaux planétaires, en se concentrant sur les contributions de l’approche de dynamique des fluides expérimentale à notre compréhension de ces processus, et en discutant de la pertinence et des limites de cette approche. Nous nous concentrons d’abord sur la dynamique des impacts planétaires, en utilisant des expériences de laboratoire pour illustrer et quantifier les processus d’impact et de cratérisation, et la dispersion de la phase métallique qui en résulte. Nous examinons ensuite l’écoulement diphasique qui suit un impact, lorsque le noyau d’un impacteur chute dans un océan de magma. Nous introduisons le modèle de thermique turbulent, qui constitue selon nous un bon modèle de référence pour l’écoulement post-impact. Nous discutons ensuite des facteurs additionnels — immiscibilité et fragmentation, inertie héritée de l’impact, force de Coriolis, sédimentation — pouvant affecter les prédictions de ce modèle. Enfin, la dernière partie de l’article est consacrée à la migration de la phase métallique à travers une partie solide du manteau.
Révisé le :
Accepté le :
Première publication :
Mots-clés : Terre primitive, formation du noyau, dynamique des fluides expérimentale
Renaud Deguen 1 ; Ludovic Huguet 1 ; Maylis Landeau 2 ; Victor Lherm 3 ; Augustin Maller 2 ; Jean-Baptiste Wacheul 4
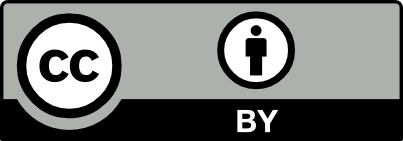
@article{CRPHYS_2024__25_S3_A22_0, author = {Renaud Deguen and Ludovic Huguet and Maylis Landeau and Victor Lherm and Augustin Maller and Jean-Baptiste Wacheul}, title = {Fluid dynamics of planetary differentiation}, journal = {Comptes Rendus. Physique}, publisher = {Acad\'emie des sciences, Paris}, year = {2024}, doi = {10.5802/crphys.227}, language = {en}, note = {Online first}, }
TY - JOUR AU - Renaud Deguen AU - Ludovic Huguet AU - Maylis Landeau AU - Victor Lherm AU - Augustin Maller AU - Jean-Baptiste Wacheul TI - Fluid dynamics of planetary differentiation JO - Comptes Rendus. Physique PY - 2024 PB - Académie des sciences, Paris N1 - Online first DO - 10.5802/crphys.227 LA - en ID - CRPHYS_2024__25_S3_A22_0 ER -
%0 Journal Article %A Renaud Deguen %A Ludovic Huguet %A Maylis Landeau %A Victor Lherm %A Augustin Maller %A Jean-Baptiste Wacheul %T Fluid dynamics of planetary differentiation %J Comptes Rendus. Physique %D 2024 %I Académie des sciences, Paris %Z Online first %R 10.5802/crphys.227 %G en %F CRPHYS_2024__25_S3_A22_0
Renaud Deguen; Ludovic Huguet; Maylis Landeau; Victor Lherm; Augustin Maller; Jean-Baptiste Wacheul. Fluid dynamics of planetary differentiation. Comptes Rendus. Physique, Online first (2024), pp. 1-45. doi : 10.5802/crphys.227.
[1] Evolution of the Earth: An introduction and overview, Treatise on Geophysics (G. Schubert, ed.), Volume 9, Elsevier, Oxford, 2015, pp. 1-9 (ISBN 978-0-444-53803-1) | DOI
[2] Asteroids were born big, Icarus, Volume 204 (2009) no. 2, pp. 558-573 | DOI
[3] Dynamical models of terrestrial planet formation, Adv. Sci. Lett., Volume 4 (2011) no. 2, pp. 325-338 | DOI
[4] Formation of the Earth’s core, Treatise on Geophysics (G. Schubert, ed.), Volume 9, Elsevier, Oxford, 2015, pp. 43-79 (ISBN 978-0-444-53803-1) | DOI
[5] Accretion of the Earth and segregation of its core, Nature, Volume 441 (2006) no. 7095, pp. 825-833 | DOI
[6] A short timescale for terrestrial planet formation from Hf/W chronometry of meteorites, Nature, Volume 418 (2002) no. 6901, pp. 949-952 | DOI
[7] Rapid accretion and early core formation on asteroids and the terrestrial planets from Hf-W chronometry, Nature, Volume 418 (2002), pp. 952-955 | DOI
[8] Broad bounds on Earth’s accretion and core formation constrained by geochemical models, Nat. Geosci., Volume 3 (2010), pp. 439-443 | DOI
[9] Metal silicate partitioning and constraints on core composition and oxygen fugacity during Earth accretion, Geochim. Cosmochim. Acta, Volume 72 (2008), pp. 574-589 | DOI
[10] Systematics of metal–silicate partitioning for many siderophile elements applied to Earth’s core formation, Geochim. Cosmochim. Acta, Volume 75 (2011) no. 6, pp. 1451-1489 | DOI
[11] Potassium in the Earth’s core?, Earth Planet. Sci. Lett., Volume 200 (2002), pp. 63-78 | DOI
[12] How much potassium is in the Earth’s core? New insights from partitioning experiments, Earth Planet. Sci. Lett., Volume 256 (2007), pp. 567-576 | DOI
[13] Potassium partitioning into molten iron alloys at high-pressure: Implications for Earth’s core, Phys. Earth Planet. Inter., Volume 160 (2007), pp. 22-33 | DOI
[14] Powering Earth’s dynamo with magnesium precipitation from the core, Nature, Volume 529 (2016) no. 7586, pp. 387-389 | DOI
[15] An early geodynamo driven by exsolution of mantle components from Earth’s core, Nature, Volume 536 (2016) no. 7616, pp. 326-328 | DOI
[16] Metal–silicate partitioning of Ni and Co in a deep magma ocean, Earth Planet. Sci. Lett., Volume 321 (2012), pp. 189-197 | DOI
[17] Core formation and core composition from coupled geochemical and geophysical constraints, Proc. Natl. Acad. Sci., Volume 112 (2015) no. 40, pp. 12310-12314 | DOI
[18] et al. High pressure metal-silicate partitioning of Ni, Co, V, Cr, Si, and O, Geochim. Cosmochim. Acta, Volume 167 (2015), pp. 177-194 | DOI
[19] Heat partitioning in metal-silicate plumes during Earth differentiation, Earth Planet. Sci. Lett., Volume 304 (2011) no. 3-4, pp. 577-586 | DOI
[20] Linking the core heat content to Earth’s accretion history, Geochem. Geophys. Geosyst., Volume 24 (2023) no. 5, e2022GC010661 | DOI
[21] Thermal evolution of the Earth: Secular changes and fluctuations of plate characteristics, Earth Planet. Sci. Lett., Volume 260 (2007), pp. 465-481 | DOI
[22] Thermal evolution of the Martian core: Implications for an early dynamo, Geology, Volume 32 (2004), pp. 97-100 | DOI
[23] Why might planets and moons have early dynamos?, Earth Planet. Sci. Lett., Volume 310 (2011) no. 3, pp. 349-359 | DOI
[24] Mixing, volatile loss and compositional change during impact-driven accretion of the Earth, Nature, Volume 427 (2004) no. 6974, pp. 505-509 | DOI
[25] The W isotope evolution of the bulk silicate Earth: Constraints on the timing and mechanisms of core formation and accretion, Earth Planet. Sci. Lett., Volume 228 (2004), pp. 109-123 | DOI
[26] Tungsten isotopic evolution during late-stage accretion: Constraints on Earth–Moon equilibration, Earth Planet. Sci. Lett., Volume 292 (2010) no. 3-4, pp. 363-370 | DOI
[27] The composition and major reservoirs of the earth around the time of the moon-forming giant impact, Treatise on Geophysics (G. Schubert, ed.), Volume 9, Elsevier, Oxford, 2015, pp. 11-42 (ISBN 978-0-444-53803-1) | DOI
[28] Early differentiation of the earth and the problem of mantle siderophile elements: A new approach, Science, Volume 253 (1991) no. 5017, pp. 303-306 | DOI
[29] Chemical evolution of the terrestrial planets, Geochim. Cosmochim. Acta, Volume 30 (1966) no. 1, pp. 41-104 | DOI
[30] Geochemistry of mantle–core differentiation at high pressure, Nature, Volume 381 (1996) no. 6584, pp. 686-689 | DOI
[31] Conditions of core formation in the Earth: Constraints from Nickel and Cobalt partitioning, Geochim. Cosmochim. Acta, Volume 69 (2005) no. 8, pp. 2141-2151 | DOI
[32] Terrestrial accretion under oxidizing conditions, Science, Volume 339 (2013) no. 6124, pp. 1194-1197 | DOI
[33] Core formation and the oxidation state of the Earth, Earth Planet. Sci. Lett., Volume 236 (2005), pp. 78-95 | DOI
[34] Accretion and core formation: Constraints from metal–silicate partitioning, Philos. Trans. R. Soc. A: Math. Phys. Eng. Sci., Volume 366 (2008) no. 1883, pp. 4339-4355 | DOI
[35] Heterogeneous accretion, composition and core–mantle differentiation of the Earth, Earth Planet. Sci. Lett., Volume 301 (2011) no. 1, pp. 31-42 | DOI
[36] Accretion and differentiation of the terrestrial planets with implications for the compositions of early-formed Solar System bodies and accretion of water, Icarus, Volume 248 (2015), pp. 89-108 | DOI
[37] Dynamique du fractionnement thermique et chimique lors de la différenciation des planètes telluriques, PhD thesis, Université de Lyon (2021) https://theses.hal.science/tel-03377150
[38] Models of the Earth’s core, Science, Volume 214 (1981) no. 4521, pp. 611-619 | DOI
[39] Core formation by giant impacts, Icarus, Volume 100 (1992) no. 2, pp. 326-346 | DOI
[40] Magma ocean formation due to giant impacts, J. Geophys. Res., Volume 98 (1993), pp. 5319-5333 | DOI
[41] Comparisons of the core and mantle compositions of earth analogs from different terrestrial planet formation scenarios, Icarus, Volume 394 (2023), 115425 | DOI
[42] Fluid dynamics of core formation, Origin of the Earth (H. E. Newsom; J. H. Jones, eds.), Oxford University Press, New York, 1990, pp. 231-249 | DOI
[43] Core formation and chemical equilibrium in the Earth—I. Physical considerations, Phys. Earth Planet. Inter., Volume 100 (1997) no. 1-4, pp. 61-79 | DOI
[44] Mechanisms of metal-silicate equilibration in the terrestrial magma ocean, Earth Planet. Sci. Lett., Volume 205 (2003) no. 3-4, pp. 239-255 | DOI
[45] Dynamics of core formation and equilibration by negative diapirism, Geochem. Geophys. Geosyst., Volume 9 (2008) no. 6, Q06011 | DOI
[46] A model of metal–silicate separation on growing planets, Earth Planet. Sci. Lett., Volume 287 (2009) no. 3-4, pp. 353-362 | DOI
[47] The influence of pressure and temperature on the metal-silicate partition coefficients of nickel and cobalt in a model C1 chondrite and implications for metal segregation in a deep magma ocean, Geochim. Cosmochim. Acta, Volume 59 (1995) no. 5, pp. 991-1002 | DOI
[48] Accretion of differentiated planetesimals to the Earth, Origin of the Earth (H. E. Newsom; J. H. Jones, eds.), 1990, pp. 29-43 | DOI
[49] Widespread magma oceans on asteroidal bodies in the early solar system, Nature, Volume 435 (2005) no. 7044, pp. 916-918 | DOI
[50] Mixing versus stirring, Annu. Rev. Fluid Mech., Volume 51 (2019), pp. 245-273 | DOI
[51] Fragmentation versus Cohesion, J. Fluid Mech., Volume 898 (2020), P1 | DOI
[52] Building the terrestrial planets: Constrained accretion in the inner solar system, Icarus, Volume 203 (2009) no. 2, pp. 644-662 | DOI
[53] The Earth’s core composition from high pressure density measurements of liquid iron alloys, Earth Planet. Sci. Lett., Volume 373 (2013), pp. 169-178 | DOI
[54] Structure, thermodynamic and transport properties of liquid MgSiO3: Comparison of molecular models and laboratory results, Geochim. Cosmochim. Acta, Volume 75 (2010), pp. 1272-1296 | DOI
[55] Transport properties of liquid metals and viscosity of the Earth’s core, Geophys. J. Int., Volume 92 (1988) no. 1, pp. 99-105 | DOI
[56] The viscosity of liquid iron at the physical conditions of the Earth’s core, Nature, Volume 392 (1998) no. 6678, pp. 805-807 | DOI
[57] Viscosity of MgSiO3 liquid at Earths mantle conditions: Implications for an early magma ocean, Science, Volume 328 (2010) no. 5979, pp. 740-742 | DOI
[58] Direct viscosity measurement of peridotite melt to lower-mantle conditions: A further support for a fractional magma-ocean solidification at the top of the lower mantle, Geophys. Res. Lett., Volume 48 (2021) no. 19, e2021GL094507 | DOI
[59] The effect of large melt fraction on the deformation behavior of peridotite, Earth Planet. Sci. Lett., Volume 246 (2006) no. 3-4, pp. 177-187 | DOI
[60] 2.18 constitutive equations, rheological behavior, and viscosity of rocks, Treatise Geophys., Volume 2 (2015), pp. 441-472
[61] The thermal conductivity of Earth’s core: A key geophysical parameter’s constraints and uncertainties, Annu. Rev. Earth Planet. Sci., Volume 46 (2018) no. 1, pp. 47-66 | DOI
[62] Transport properties of silicate melts, Rev. Geophys., Volume 53 (2015) no. 3, pp. 715-744 | DOI
[63] Interfacial tension of Fe–Si liquid at high pressure: Implications for liquid Fe-alloy droplet size in magma oceans, Phys. Earth Planet. Inter., Volume 202 (2012), pp. 1-6 | DOI
[64] Magma oceans and primordial mantle differentiation, Treatise on Geophysics (G. Schubert, ed.), Volume 9, Elsevier, Oxford, 2015, pp. 81-104 (ISBN 978-0-444-53803-1) | DOI
[65] Turbulent mixing of metal and silicate during planet accretion — And interpretation of the Hf–W chronometer, Earth Planet. Sci. Lett., Volume 295 (2010), pp. 177-186 | DOI
[66] Experiments on turbulent metal-silicate mixing in a magma ocean, Earth Planet. Sci. Lett., Volume 310 (2011), pp. 303-313 | DOI
[67] Collisional stripping of Mercury’s mantle, Icarus, Volume 74 (1988), pp. 516-528 | DOI
[68] Accretion of the terrestrial planets and the Earth–Moon system, Origin of the Earth and Moon (R. M. Canup; K. Righter, eds.), University of Arizona Press, Tucson, AZ, 2000, pp. 113-129 | DOI
[69] Making the Moon from a fast-spinning Earth: A giant impact followed by resonant despinning, Science, Volume 338 (2012) no. 6110, pp. 1047-1052 | DOI
[70] Melting and mixing states of the Earth’s mantle after the Moon-forming impact, Earth Planet. Sci. Lett., Volume 427 (2015), pp. 286-295 | DOI
[71] Immediate origin of the moon as a post-impact satellite, Astrophys. J. Lett., Volume 937 (2022) no. 2, L40 | DOI
[72] Differentiated planetesimal impacts into a terrestrial magma ocean: fate of the iron core, Earth Planet. Sci. Lett., Volume 448 (2016), pp. 24-33 | DOI
[73] Direct numerical simulation of an iron rain in the magma ocean, J. Geophys. Res., Volume 115 (2010), B01404 | DOI
[74] A re-evaluation of metal diapir breakup and equilibration in terrestrial magma oceans, Earth Planet. Sci. Lett., Volume 313 (2012), pp. 105-114 | DOI
[75] Turbulent metal-silicate mixing, fragmentation, and equilibration in magma oceans, Earth Planet. Sci. Lett., Volume 391 (2014), pp. 274-287 | DOI
[76] Metal-silicate mixing by large Earth-forming impacts, Earth Planet. Sci. Lett., Volume 564 (2021), 116888 | DOI
[77] Rayleigh–Taylor instability in impact cratering experiments, J. Fluid Mech., Volume 937 (2022), A20 | DOI
[78] Influence of planetary rotation on metal-silicate mixing and equilibration in a magma ocean, Phys. Earth Planet. Inter., Volume 352 (2024), 107168 | DOI
[79] Impact Cratering: A Geologic Process (H. J. Melosh, ed.), Oxford Monographs on Geology and Geophysics, No. 11, Oxford University Press, New York, 1989, p. 253
[80] The scaling of impact processes in planetary sciences, Annu. Rev. Earth Planet. Sci., Volume 21 (1993), pp. 333-373 | DOI
[81] et al. Validation of numerical codes for impact and explosion cratering: Impacts on strengthless and metal targets, Meteorit. Planet. Sci., Volume 43 (2008) no. 12, pp. 1917-1938 | DOI
[82] Degree of impactor fragmentation under collision with a regolith surface–Laboratory impact experiments of rock projectiles, Meteorit. Planet. Sci., Volume 49 (2014) no. 1, pp. 69-79 | DOI
[83] Crater depth in fluid impacts, J. Appl. Phys., Volume 37 (1966) no. 4, pp. 1798-1808 | DOI
[84] Velocity field and cavity dynamics in drop impact experiments, J. Fluid Mech., Volume 962 (2023), A21 | DOI
[85] Drop fragmentation at impact onto a bath of an immiscible liquid, Phys. Rev. Lett., Volume 110 (2013) no. 26, 264503 | DOI
[86] Condition for metal fragmentation during Earth-forming collisions, Phys. Earth Planet. Inter., Volume 352 (2024), 107199 | DOI
[87] Laboratory experiments on the breakup of liquid metal diapirs, Earth Planet. Sci. Lett., Volume 403 (2014), pp. 236-245 | DOI
[88] Experiments on fragmentation and thermo-chemical exchanges during planetary core formation, Phys. Earth Planet. Inter., Volume 276 (2018), pp. 134-144 | DOI
[89] On impact with a liquid surface, Proc. R. Soc. Lond., Volume 34 (1883) no. 220-223, pp. 217-230 | DOI
[90] V. Impact with a liquid surface, studied by the aid of instantaneous photography, Philos. Trans. R. Soc. Lond. Ser. A-Contain. Pap. Math. Phys. Character., Volume 189 (1897), pp. 137-148 | DOI
[91] IV. Impact with a liquid surface studied by the aid of instantaneous photography. Paper II, Philos. Trans. R. Soc. Lond. Ser. A-Contain. Pap. Math. Phys. Character., Volume 194 (1900) no. 252-261, pp. 175-199 | DOI
[92] Initial pressure, initial flow velocity, and the time dependence of crater depth in fluid impacts, J. Appl. Phys., Volume 38 (1967) no. 10, pp. 3935-3940 | DOI
[93] The impact of drops on liquid surfaces and the underwater noise of rain, Annu. Rev. Fluid Mech., Volume 25 (1993) no. 1, pp. 577-602 | DOI
[94] Splash formation by spherical drops, J. Fluid Mech., Volume 427 (2001), pp. 73-105 | DOI
[95] On some common features of drop impact on liquid surfaces, Phys. Fluids, Volume 16 (2004) no. 5, pp. 1349-1365 | DOI
[96] Crater evolution after the impact of a drop onto a semi-infinite liquid target, Phys. Rev. E, Volume 82 (2010) no. 3, 036319 | DOI
[97] Regimes during liquid drop impact on a liquid pool, J. Fluid Mech., Volume 768 (2015), pp. 492-523 | DOI
[98] Rayleigh–Taylor instability in drop impact experiments, Phys. Rev. Fluids, Volume 6 (2021) no. 11, 110501 | DOI
[99] Deep pool water-impacts of viscous oil droplets, Soft Matter, Volume 15 (2019) no. 23, pp. 4629-4638 | DOI
[100] Fragmentation of a liquid metal droplet falling in a water pool, Phys. Fluids, Volume 33 (2021) no. 10, 103315 | DOI
[101] et al. Oblique drop impact onto a deep liquid pool, Phys. Rev. Fluids, Volume 2 (2017) no. 8, 083602 | DOI
[102] Planetary impacts: Scaling of crater depth from subsonic to supersonic conditions, J. Geophys. Res.: Planets, Volume 128 (2023) no. 8, e2023JE007823 | DOI
[103] Sonic eddy-a model for compressible turbulence, AIAA J., Volume 30 (1992) no. 1, pp. 101-104 | DOI
[104] Compressibility effects in a turbulent annular mixing layer. Part 1. Turbulence and growth rate, J. Fluid Mech., Volume 421 (2000), pp. 229-267 | DOI
[105] A study of compressibility effects in the high-speed turbulent shear layer using direct simulation, J. Fluid Mech., Volume 451 (2002) no. 1, pp. 329-371 | DOI
[106] On density effects and large structure in turbulent mixing layers, J. Fluid Mech., Volume 64 (1974), pp. 775-816 | DOI
[107] Understanding oblique impacts from experiments, observations, and modeling, Annu. Rev. Earth Planet. Sci., Volume 28 (2000) no. 1, pp. 141-167 | DOI
[108] Experimental studies of oblique impact, Lunar and Planetary Science Conference, 9th, Houston, Tex., March 13–17, 1978, Proceedings. Volume 3. (A79-39253 16-91) New York, Volume 9, Pergamon Press, Inc., 1978, pp. 3843-3875
[109] Melt production in oblique impacts, Icarus, Volume 145 (2000) no. 1, pp. 252-261 | DOI
[110] Regimes of drop morphology in oblique impact on deep fluids, J. Fluid Mech., Volume 543 (2005), pp. 303-331 | DOI
[111] Effect of impingement angle on the outcome of single water drop impact onto a plane water surface, Exp. Fluids, Volume 44 (2008), pp. 331-339 | DOI
[112] A laboratory model for oblique planetary impacts (in preparation)
[113] Heat convection and buoyancy effects in fluids, Q. J. R. Met. Soc., Volume 80 (1954), pp. 339-358 | DOI
[114] Turbulent gravitational convection from maintained and instantaneous sources, Proc. R. Soc. Lond. Ser. A, Math. Phys. Sci., Volume 234 (1956), pp. 1-23 | DOI
[115] Experiments on convection of isolated masses of buoyant fluid, J. Fluid Mech. Dig. Arch., Volume 2 (1957) no. 06, pp. 583-594 | DOI
[116] The motion in and around isolated thermals, Q. J. R. Meteorol. Soc., Volume 85 (1959) no. 364, pp. 144-151 | DOI
[117] Buoyant plumes and thermals, Annu. Rev. Fluid Mech., Volume 1 (1969) no. 1, pp. 29-44 | DOI
[118] Entrainment in resolved, dry thermals, J. Atmosph. Sci., Volume 76 (2019) no. 12, pp. 3785-3801 | DOI
[119] Fluid dynamics of Earth’s core: geodynamo, inner core dynamics, core formation, Fluid Mechanics of Planets and Stars (M. Le Bars; D. Lecoanet, eds.), Springer, 2020, pp. 129-212 | DOI
[120] Entrainment in resolved, dry thermals, J. Atmosph. Sci., Volume 76 (2019) no. 12, pp. 3785-3801 | DOI
[121] A numerical and analytical study of the effect of aspect ratio on the behavior of a round thermal, Environ. Fluid Mech., Volume 15 (2015), pp. 85-108 | DOI
[122] What controls the entrainment rate of dry buoyant thermals with varying initial aspect ratio?, J. Atmos. Sci., Volume 80 (2023) no. 11, pp. 2711-2728 | DOI
[123] On the internal vorticity and density structures of miscible thermals, J. Fluid Mech., Volume 722 (2013), R5 | DOI
[124] Experiments on the fragmentation of a buoyant liquid volume in another liquid, J. Fluid Mech., Volume 749 (2014), pp. 478-518 | DOI
[125] On the self-similarity of turbulent thermals, J. Fluid Mech. (in preparation)
[126] The motion of a turbulent thermal in the presence of background rotation, J. Atmos. Sci., Volume 51 (1994) no. 13, pp. 1989-1994 | DOI
[127] Thermals with background rotation and stratification, J. Fluid Mech., Volume 259 (1994), pp. 265-280 | DOI
[128] Effects of particle size and background rotation on the settling of particle clouds, Phys. Rev. Fluids, Volume 7 (2022) no. 12, 124302 | DOI
[129] Experiments on turbulent metal-slicate mixing in a magma ocean, Earth Planet. Sci. Lett., Volume 310 (2011) no. 3-4, pp. 303-313 | DOI
[130] Particle clouds in homogeneous and stratified environments, J. Fluid Mech., Volume 489 (2003), pp. 29-54 | DOI
[131] Fall and fragmentation of liquid metal in a viscous fluid, Phys. Rev. Fluids, Volume 2 (2017) no. 9, 090507 | DOI
[132] On spray formation, J. Fluid Mech., Volume 498 (2004), pp. 73-111 | DOI
[133] Fundamentals of the hydrodynamic mechanism of splitting in dispersion processes, AIChE. J., Volume 1 (1955) no. 3, pp. 289-295 | DOI
[134] Dynamics and stability of an iron drop falling in a magma ocean, Phys. Earth Planet. Inter., Volume 289 (2019), pp. 75-89 | DOI
[135] Étude de la fragmentation de métal liquide en chute libre dans un environnement visqueux: application à la formation des planètes— Theses. fr., PhD thesis, Aix-Marseille (2019)
[136] On the motion of turbulent thermals, J. Fluid Mech., Volume 61 (1973) no. 03, pp. 541-552 | DOI
[137] Core merging and stratification following giant impact, Nat. Geosci., Volume 9 (2016) no. 10, pp. 786-789 | DOI
[138] Crystal settling in a vigorously convecting magma chamber, Nature, Volume 332 (1988) no. 6164, pp. 534-536 | DOI
[139] A fluid-dynamical study of crystal settling in convecting magmas, J. Petrol., Volume 30 (1989) no. 6, pp. 1471-1500 | DOI
[140] Sedimentation of particles in a vigorously convecting fluid, Phys. Rev. E, Volume 80 (2009) no. 4, 046324 | DOI
[141] Entrainment from a bed of particles by thermal convection, Earth Planet. Sci. Lett., Volume 120 (1993) no. 3-4, pp. 387-393 | DOI
[142] Dynamics of a terrestrial magma ocean under planetary rotation: A study in spherical geometry, Earth Planet. Sci. Lett., Volume 513 (2019), pp. 81-94 | DOI
[143] On the fate of impact-delivered metal in a terrestrial magma ocean, Earth Planet. Sci. Lett., Volume 554 (2021), 116680 | DOI
[144] Early history of the Earth, Earth Science and Meteorites (J. Geiss; E. D. Goldberg, eds.), North-Holland Pub. Co., Amsterdam, 1963, pp. 113-129
[145] Metal-silicate fractionation in the growing Earth: Energy source for the terrestrial magma ocean, J. Geophys. Res.: Solid Earth, Volume 91 (1986) no. B9, pp. 9231-9238 | DOI
[146] The Earth’s core formation due to the Rayleigh–Taylor instability, Icarus, Volume 69 (1987) no. 2, pp. 239-248 | DOI
[147] Dynamics of laboratory diapir and plume models, J. Geophys. Res., Volume 80 (1975) no. 5, pp. 705-717 | DOI
[148] et al. Iron diapirs entrain silicates to the core and initiate thermochemical plumes, Nat. Commun., Volume 9 (2018) no. 1, 71 | DOI
[149] Hydrodynamic and Hydromagnetic Stability, Dover Publications, Inc., Mineola, New York, 2013
[150] The non-linear initiation of diapirs and plume heads, Phys. Earth Planet. Inter., Volume 101 (1997), pp. 119-130 | DOI
[151] Bubbles, Drops, and Particles, Dover Publications, Inc., Mineola, New York, 2005
[152] Compositional and thermal equilibration of particles, drops, and diapirs in geophysical flows, Geochem. Geophys. Geosyst., Volume 12 (2011) no. 10, Q10014 | DOI
[153] Heat partitioning in terrestrial planets during core formation by negative diapirism, Earth Planet. Sci. Lett., Volume 290 (2010) no. 1-2, pp. 13-19 | DOI
[154] Experiments on metal–silicate plumes and core formation, Philos. Trans. R. Soc. A: Math. Phys. Eng. Sci., Volume 366 (2008) no. 1883, pp. 4253-4271 | DOI
[155] On the mechanics of igneous diapirism, stoping, and zone melting, Am. J. Sci., Volume 282 (1982) no. 6, pp. 808-855 | DOI
[156] The effects of a strongly temperature-dependent viscosity on slow flow past a hot sphere, J. Fluid Mech., Volume 124 (1982), pp. 1-26 | DOI
[157] The effects of a strongly temperature-dependent viscosity on Stokes’s drag law: Experiments and theory, J. Fluid Mech., Volume 159 (1985), pp. 459-476 | DOI
[158] Diapirism in the Earth’s mantle: experiments on the motion of a hot sphere in a fluid with temperature dependent viscosity, J. Volcanol. Geotherm. Res., Volume 16 (1983), pp. 221-245 | DOI
[159] Mission to Earth’s core—a modest proposal, Nature, Volume 423 (2003) no. 6937, pp. 239-240 | DOI
[160] Dikes vs. diapirs in viscoelastic rock, Earth Planet. Sci. Lett., Volume 117 (1993) no. 3-4, pp. 653-670 | DOI
[161] Experiments on buoyancy-driven crack around the brittle–ductile transition, Earth Planet. Sci. Lett., Volume 304 (2011) no. 3-4, pp. 337-346 | DOI
[162] Experiments on gravitational phase separation of binary immiscible fluids, J. Fluid Mech., Volume 591 (2007), pp. 289-319 | DOI
[163] Percolation of core melts at lower mantle conditions, Science, Volume 280 (1998) no. 5366, pp. 1059-1061 | DOI
[164] Core formation in planetesimals triggered by permeable flow, Nature, Volume 422 (2003) no. 6928, pp. 154-157 | DOI
[165] Interconnectivity of Fe–O–S liquid in polycrystalline silicate perovskite at lower mantle conditions, Phys. Earth Planet. Inter., Volume 161 (2007) no. 3-4, pp. 170-176 | DOI
[166] Percolative core formation in planetesimals, Earth Planet. Sci. Lett., Volume 273 (2008) no. 1-2, pp. 132-137 | DOI
[167] Metal segregation in planetesimals: Constraints from experimentally determined interfacial energies, Earth Planet. Sci. Lett., Volume 518 (2019), pp. 40-52 | DOI
[168] Metal-silicate segregation in deforming dunitic rocks, Geochem. Geophys. Geosyst., Volume 7 (2006) no. 2, Q02001 | DOI
[169] Textural evolution of metallic phases in a convecting magma ocean: A 3D microtomography study, Phys. Earth Planet. Inter., Volume 319 (2021), 106771 | DOI
[170] Differentiation time scales of small rocky bodies, Icarus, Volume 390 (2023), 115294 | DOI
[171] A multiphase model of core formation, Geophys. J. Int., Volume 181 (2010) no. 1, pp. 198-220 | DOI
[172] Thermal evolution and differentiation of planetesimals and planetary embryos, Icarus, Volume 217 (2012) no. 1, pp. 339-354 | DOI
[173] Magma ascent in planetesimals: Control by grain size, Earth Planet. Sci. Lett., Volume 507 (2019), pp. 154-165 | DOI
[174] Formation, stratification, and mixing of the cores of Earth and Venus, Earth Planet. Sci. Lett., Volume 174 (2017), pp. 375-386 | DOI
[175] Versuche zum Wärmeübergang bei natürlicher Konvektion, Chemie Ingenieur Technik, Volume 28 (1956) no. 3, pp. 175-180 | DOI
[176] Pseudosteady-state natural convection inside spheres, J. Heat Transfer, Volume 97 (1975) no. 1, pp. 54-59 | DOI
[177] Pseudosteady-state natural convection heat transfer inside spheres, Int. J. Heat Mass Transfer, Volume 32 (1989) no. 11, pp. 2047-2053 | DOI
Cité par Sources :
Commentaires - Politique