1 Introduction
Initiation of translation on a subset of eukaryotic mRNAs occurs by a non-canonical 5′-end-independent process of ribosomal attachment that is mediated by an internal ribosomal entry site (IRES) in the mRNA. IRESs are functionally defined by their ability to promote translation of the downstream cistron in a dicistronic mRNA independently of translation of the upstream cistron, and they occur in various cellular and viral mRNAs [1]. Related viruses may contain similar IRESs, but otherwise most IRESs differ from one another in terms of length, sequence and structure. Recent research into initiation mediated by two groups of unrelated IRESs, exemplified by hepatitis C virus (HCV) and cricket paralysis virus (CrPV), respectively, has revealed two distinct mechanisms of IRES-mediated initiation that both involve precise, factor-independent binding to ribosomes, and that are remarkably different from the canonical 5′-end- dependent mechanism of initiation.
1.1 The canonical mechanism of translation initiation
Initiation on most eukaryotic mRNAs occurs by the scanning mechanism, in which ribosomal preinitiation complexes bind to the capped 5′-end of mRNA and scan downstream to locate the initiation codon [2]. This process is mediated by at least ten eukaryotic initiation factors (eIFs) and requires that the 80S ribosome first be separated into 40S and 60S subunits. The multisubunit eIF3 plays the principal role in ribosomal dissociation and anti-association [3], but for this, it additionally requires the presence of either mRNA that is competent to bind to the 40S subunit, or the aminoacylated initiator tRNA (Met-tRNAMeti)/eIF2-GTP ternary complex [4,5]. Dissociation by eIF3 in the presence of RNA cofactor is enhanced by eIF1 and eIF1A [4]. The ternary complex stabilizes association of other factors on the 40S subunit, forming a 43S preinitiation complex. The heterotrimeric eIF4F mediates attachment of 43S complexes to the 5′-proximal region of mRNA. The mechanism by which it does so probably involves binding of its eIF4E subunit to the 5′-terminal m7G ‘cap’, unwinding of adjacent mRNA by the eIF4A (RNA helicase) subunit, interaction of the eIF4G subunit with eIF3, and interaction of both eIF3 and eIF4G with the mRNA. This process may be enhanced by the poly(A) binding protein, which binds to eIF4G and to the 3′-poly(A) tail of mRNAs, and by eIF4B. The 43S complex then scans downstream until it encounters an AUG triplet, which acts as the initiation codon, leading to formation of a 48S complex in which the AUG codon and the anticodon of Met-tRNAMeti are base-paired in the ribosomal P site. Initiation codon selection is primarily determined by complementarity to this anticodon, and is influenced by the nucleotide context of the initiation codon. AUG triplets that diverge from the optimum context AccG (in which the bold residues are the most important) may be bypassed, leading to initiation downstream [6]. The principal role in maintaining the fidelity of initiation codon selection is played by eIF1, which discriminates against initiation at non-AUG triplets and at AUG triplets with suboptimal context [7]. Following 48S complex formation at the initiation codon, eIF5 induces hydrolysis of eIF2-bound GTP, eIF2-GDP is released and Met-tRNAMeti is left in the ribosomal P site. Finally, eIF5B-GTP displaces the remaining factors from the 40S subunit and mediates its joining with the 60S subunit to form an 80S ribosome, which becomes competent to enter the elongation phase of translation following hydrolysis of GTP and release of eIF5B-GDP [5,8].
1.2 Comparisons between translation initiation in eukaryotes and in prokaryotes
The canonical mechanism of initiation in eukaryotes resembles the initiation process in prokaryotes in that both begin with separated subunits, both require a unique initiator tRNA that is specifically bound by a dedicated factor (IF2 in prokaryotes) to the P site of the small ribosomal subunit while elongator tRNAs are excluded, and both proceed via an obligatory intermediate complex comprising the small subunit bound to mRNA and initiator tRNA. There are also significant differences between these processes. Initiation in prokaryotes involves only three factors, and prokaryotic mRNAs can bind directly to the 30S ribosomal subunit without the involvement of initiation factors or the prior recruitment of initiator tRNA. Prokaryotic mRNA binds in a cleft between the head and platform of the 30S subunit [9] and is stabilized by base-pairing between the short purine-rich Shine–Dalgarno (SD) sequence, 5–7 nt. upstream of the initiation codon, and a complementary anti-SD sequence at the 3′-end of 16S ribosomal RNA (rRNA). This sequence is absent from the equivalent eukaryotic 18S rRNA. The SD sequence and the initiation codon must occur in an unstructured region of mRNA to allow initiation to occur [10] presumably because the prokaryotic translation apparatus is unable to direct a RNA helicase equivalent to eukaryotic eIF4A to unwind secondary structure in the vicinity of the ribosome binding site.
The influential view that eukaryotic ribosomes can initiate translation only by end-dependent attachment to mRNA and are incapable of binding directly to it at an internal position [6,11] was overturned by reports that ∼450 nt.-long IRESs in encephalomyocarditis virus (EMCV) and poliovirus mRNAs mediated ribosomal attachment independently of the 5′-end [12,13]. Neither of these IRESs can bind directly to 40S subunits in the absence of initiation factors: accurate 48S complex formation on the EMCV IRES required the same factors as initiation on capped mRNA, except for eIF4E and the large amino-terminal domain of eIF4G to which it binds [14,15]. This domain is cleaved from eIF4G by a virus-encoded protease during poliovirus infection, which shuts off host cell protein synthesis but does not abolish initiation on EMCV and poliovirus IRESs. The central domain of mammalian eIF4G binds specifically to the EMCV IRES, mediating recruitment of the 40S subunit in an ATP-dependent manner and leading to 48S complex formation at the authentic initiation codon [15,16].
Two groups of viral IRESs have subsequently been identified that are structurally unrelated to the EMCV and poliovirus IRESs. As described here, they use unrelated mechanisms of initiation that differ both from canonical end-dependent and from EMCV IRES-mediated mechanisms of initiation. These novel mechanisms both involve factor-independent binding of mRNA to the 40S subunit; however, the binding mechanisms are fundamentally different from the mechanism by which 30S subunits bind to prokaryotic mRNA.
2 Internal initiation by factor-independent binding of ribosomes to the initiation codon
2.1 The structure of hepatitis C virus and pestivirus IRESs
The 5′NTRs of Hepatitis C virus (HCV), GB virus B (GBV-B) and of pestiviruses such as bovine viral diarrhea virus (BVDV) and classical swine fever virus (CSFV) all contain an IRES [17–22]. The 341 nt.-long HCV 5′NTR consists of four major structural domains, designated I to IV (Fig. 1). Domain III is the most complex, comprising a pseudoknot, several helices, and hairpin domains IIIa–IIIf. Domains IIIa–IIIc and IIIe–IIIf form four-way helical junctions, folding of which is dependent on physiological concentrations of divalent metal ions [23–25]. The 5′NTRs of HCV, GBV-B and pestiviruses contain conserved sequences and have recognizably similar, but not identical secondary structures [26,27]. Sequence differences include large insertions (GBV-B contains two additional hairpins in domain II, and pestivirus 5′NTRs contain an additional hairpin (IIId2) in domain III), small insertions and substitutions that, for example, result in the equivalent of domain IV in pestiviruses being unstructured [28]. Nevertheless, key structural and functional elements are conserved in all HCV-like IRESs, and fundamental aspects of the mechanism of initiation on them are identical.
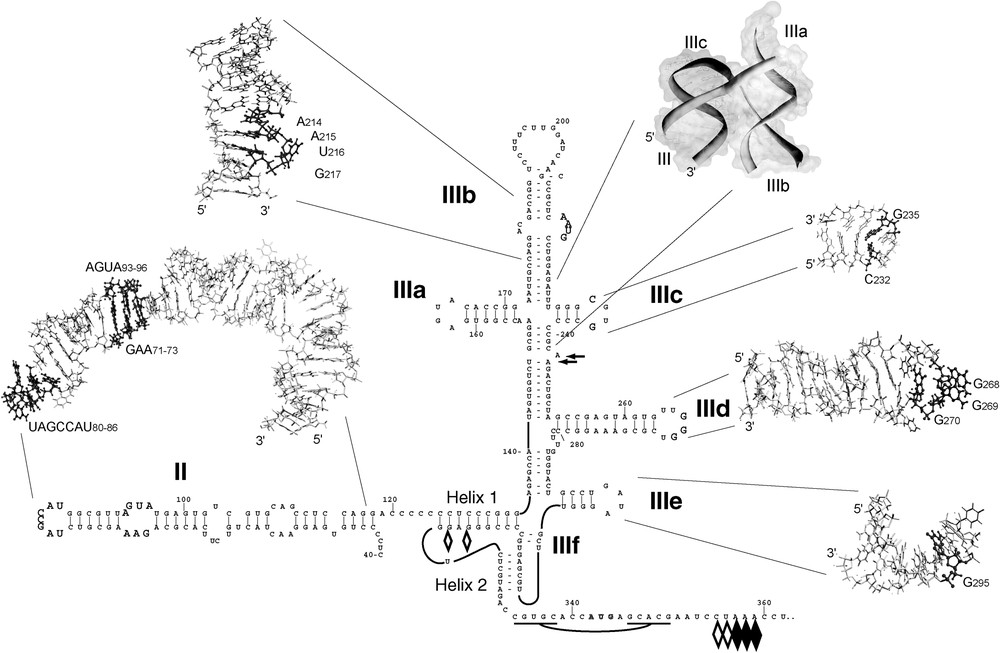
Structure of domains II, III, and IV of HCV-like IRESs. Sequences flanking the initiation codon are base paired as indicated to form domain IV in HCV but not in BVDV or CSFV. BVDV and CSFV contain two hairpins (IIId1 and IIId2) at an analogous position to HCV IIId. The nomenclature of helices in the pseudoknot and of domains is as in [1,35]. The toeprints detected at the leading edge of bound eIF3 [38,47,48] are indicated by arrows. The toeprints at the leading edge of 40S subunits and in the pseudoknot in binary HCV IRES:40S subunit complexes are indicated by open diamonds, and toeprints at the leading edge of 48S complexes formed on inclusion of eIF2-GTP/ Met-tRNAMeti with 40S subunits are indicated by filled diamonds. Structures of domains and subdomains of the HCV IRES were visualized using YASARA, and functionally important nucleotides are shaded dark grey. Domain II (PDB accession code 1P5P) is annotated to show nucleotides that are conserved in HCV-like IRESs, a fragment of domain IIIb (PDB 1KP7) is annotated to show a conserved sequence motif that is bound by eIF3 [38,49], the IIIabc four-way junction (PDB 1KH6) shows the stacking and orientation of helices, domain IIIc (PDB 1R4H) is annotated to show the buckled C-G base-pair of the tetraloop, domain IIId (PDB 1F84) is annotated to show GGG268–270 and domain IIIe (PDB 1F85) is annotated to show G295, which bind directly to the 40S subunit [44,49,50].
HCV, BVDV and CSFV IRESs extend from the 5′-border of domain II to the initiation codon: truncation or deletion of domain II significantly impairs but does not abolish function [29–32]. The coding region adjacent to the 3′-border of the IRES enhances its activity, but can be replaced by heterologous coding sequences as long as they are A-rich and have little tendency to form secondary structure [33,34]. Sequences that increase the degree of secondary structure flanking the initiation codon reduce the efficiency of initiation [33,35–37].
The structure of the HCV IRES has been determined at ∼20-Å resolution by cryo-electron microscopy (cryo-EM) [38] and at higher resolution for some of its structural components by X-ray crystallography and NMR. These include domain II [39], the four-way IIIabc junction [40], part of domain IIIb [41], domain IIIc [42], and domains IIId and IIIe [43,44].
2.2 Factor requirements for initiation of translation on HCV-like IRESs
Internal entry of ribosomes on the HCV IRES leads to the initiation codon being placed at or in the immediate vicinity of the P site: ribosomes are unable to recognize AUG triplets introduced only 7 nt. upstream of the initiation codon or 8 nt. downstream of an inactivated initiation codon, indicating that they cannot scan from the initial point of attachment [32,34,45].
The minimum set of factors required for assembly of 48S complexes on BVDV, CSFV and HCV IRESs was determined by in vitro reconstitution of this process using purified translation components [46–48]. Formation of 48S complexes was assayed using sucrose density gradient centrifugation, and the position of complexes on the RNA was assayed using primer extension inhibition (‘toeprinting’). In this assay, extension of a primer annealed to mRNA downstream of a ribosomal binding site by reverse transcriptase is arrested by the bound ribosome, yielding toeprints at characteristic positions 15–17 nt. downstream of the +1 nucleotide of the triplet in the P site. These studies showed that 40S subunits bind directly and specifically to HCV-like IRESs without the involvement of initiation factors, in such a way that the initiation codon is placed in the immediate vicinity of the P site. At the time of its discovery [48], the ability of the IRES to bind directly to the 40S subunit in this way was unprecedented. Binding of the eIF2-ternary complex to the IRES-bound 40S subunit leads to formation of a 48S complex with correct codon–anticodon base-pairing, and induces a slight rearrangement of the initiation codon and coding region on the 40S subunit relative to their position in the binary IRES/40S subunit complex. The yield of 48S complex assembled on the CSFV IRES was not influenced by eIF1 and eIF1A, but these factors also caused minor changes in this region of mRNA on the 40S subunit that are suggestive of conformational change in the 48S complex [46]. We have suggested elsewhere that eIF1 alone may induce conformational changes in the 40S subunit [51].
eIF3 also binds specifically to the IRES [47,48] to a site that has been localized to the IIIabc region by footprinting [38,49]. Disruption of this region by mutation impairs or abrogates binding of eIF3 and leads to loss of IRES function [38,48,52]. eIF3 may stabilize assembled 48S complexes, but it is not essential for their formation. However, it is required for joining of a 60S subunit to the IRES-bound 48S complex [15]. The mechanism of subunit joining on HCV-like IRESs has not yet been established, but likely involves both eIF5 and eIF5B. A possible function of eIF3 at this stage may be to stabilize the ribosomal complex containing Met-tRNAMeti in the P site following dissociation of eIF2-GDP, after eIF5-induced hydrolysis of eIF2-bound GTP and before subunit joining [5]. The order of binding of eIF3 and the IRES to a 40S subunit before recruitment of the eIF2 ternary complex has not been conclusively established, but our recent observations that eIF3 alone does not bind stably to 40S subunits and that their stable association is mRNA-dependent [4], taken together with the fact that the IRES binds stably to 40S subunits in a factor-independent manner [48] are consistent with the suggestion that the IRES binds to 40S subunits before eIF3 [31].
A striking property of this initiation mechanism is that it has no requirement for eIF4F, any of its component subunits, eIF4B or ATP hydrolysis. It is consequently resistant to inhibition by poliovirus-mediated cleavage of eIF4G and by dominant negative eIF4A mutants [18,22,47]. Indeed, the inhibitory effects on IRES-mediated translation of sequestering the HCV initiation codon in stable secondary structure or of introducing a hairpin immediately downstream of the CSFV initiation codon [35,37] (neither of which would impede a conventionally scanning ribosome) both strongly suggest that the IRES/preinitiation complex is incapable of involving the eIF4 group of factors to unwind mRNA secondary structure at the site of ribosome binding. By contrast, this is considered to be a characteristic step in 5′-end-dependent attachment of ribosomes to mRNA.
Some cellular RNA-binding proteins, such as PTB, La, hnRNP-L and NSAP-1 bind to sites flanking the HCV initiation codon, and it has been suggested that they may act by unwinding domain IV or maintaining it in an unfolded state [53–56]. However, they are clearly not sufficient to overcome inhibition of IRES function by even minor sequence changes that increase the stability of domain IV. Moreover, the specificity of binding of some of these proteins and their functional importance in initiation on HCV-like IRESs have been disputed [57,58].
2.3 The mechanism of ribosome binding and assembly on HCV-like IRESs
The most remarkable and distinctive aspect of initiation on HCV-like IRESs is their ability to bind specifically and stably to 40S subunits in the complete absence of initiation factors in a manner that leads to and is required for formation of elongation-competent 80S ribosomes. It is thus of obvious interest to determine the mechanism of ribosomal binding to these IRESs. Toeprinting and deletion analyses indicate that binding of 40S subunits to HCV and pestivirus IRESs involves contacts at multiple sites [47,48]; these have been mapped in detail on HCV and CSFV IRESs by chemical and enzymatic footprinting [44,49,50,59]. The sites of interaction of 40S subunits with these IRESs are similar and correspond to the regions of greatest sequence conservation. They include nucleotides near the apex of domain II, in domains IIId (or the equivalent CSFV IIId1) and IIIe, in the pseudoknot, and flanking the initiation codon. These sites of interaction are consistent with cryo-EM studies of IRES/40S subunit complexes [60], which, however, contradict a report that the 40S subunit also binds to the IIIabc region [49] and instead indicate that it extends away from the 40S subunit. Although mutational analysis suggest that the highly conserved IIIa loop is strongly required for HCV IRES function [49], similar analyses indicated that it is much less important for CSFV IRES function [30,59]. Domain IIIc has a novel tetraloop fold, in which the first and fourth nucleotides are base-paired, and the bases of the second and third nucleotides protrude into the solvent [42]. The sequence of nucleotides in the stem is critical for IRES function, whereas the identity of the protruding loop residues is less important [42,61]. Deletion of domain IIIc abrogated binding of eIF3 but not of 40S subunits to the HCV IRES [38,48]. There is no consensus yet concerning the extent of the overlap between binding sites for eIF3 and the 40S subunit on the IRES.
The number of nucleotides flanking the initiation codon that the ribosome protects from cleavage/modification correspond to the length of the mRNA-binding channel of the 40S subunit, and this observation suggests that other binding determinants in the IRES contact the 40S subunit outside this channel. Cryo-EM analysis indicated that the IRES binds primarily to the solvent side of the 40S subunit, near the entrance to this channel, and makes additional contacts with the E site [60]. Mutational analyses indicated that domain II, helix 2 of the pseudoknot or nucleotides flanking the initiation codon are not required for high-affinity binding of the IRES to the 40S subunit [31,48–50,59,62]. As we have emphasized elsewhere [1,26,63], the lack of correlation between elements of the IRES required for high-affinity binding and for function indicates clearly that the initiation mechanism on these IRESs cannot be described simply in terms of direct binding of the IRES to the 40S subunit, and that the IRES plays additional, more sophisticated roles in initiation. The importance of the pseudoknot for IRES function was recognized at an early stage [64,65]. Helix 1 of the pseudoknot is required for stable binding of the IRES to the 40S subunit, whereas mutations in helix 2 do not prevent ribosomal attachment to the IRES, but impair stable docking of the initiation codon and flanking sequences in the mRNA-binding channel of the 40S subunit and thus lead to loss of function.
Footprinting implicates the conserved apex of domain IIId in HCV and CSFV IRESs in contacting the 40S subunit directly, and mutational analyses have repeatedly confirmed its importance for ribosomal binding [39,49,50,66] whereas the highly conserved domain IIIe appears to be more important for ribosomal binding to the HCV IRES than to pestiviruses [29,44,59,67]. The helical stem of HCV domain IIId is interrupted by an asymmetric loop in which the Watson–Crick faces of A260 and A274–276 are exposed to the minor groove [44]; however, this distinctive structure is not essential for IRES function [31,66] and is not present in pestivirus IRESs. The apical loop of domain IIId contains three residues, G266, G267 and G268, that are critical determinants of ribosomal binding. The HCV domain IIIe loop has a novel tetraloop fold in which G295, A296 and U297 form an array of exposed bases. G295 contacts the 40S subunit directly [44,49].
It is likely that binding of the IRES occurs in at least two (and probably more) stages: accommodation of the sequence flanking the initiation codon in the mRNA-binding channel of the 40S subunit follows and is dependent on initial high-affinity binding to the 40S subunit of a core element containing domains IIId and IIIe, and helix 1 of the pseudoknot. Toeprinting indicated that this second step is dependent on the integrity of pseudoknot helix 2 [48] and on domain II, because in its absence, the initiation codon and adjacent coding region do not bind stably in the mRNA-channel [50].
Cryo-EM [60] confirmed that domain II directly influences binding of mRNA downstream of the pseudoknot to the 40S subunit. The site to which domain II binds partially overlaps the 40S subunit E (exit) site, from which deacylated tRNA is released during elongation, and this IRES/40S subunit contact closes the mRNA binding channel from the intersubunit side, trapping mRNA. The intact IRES induces multiple conformational changes in the 40S subunit that include rearrangement of the mRNA binding channel and rotation of the head relative to the body. These changes are dependent on domain II. The interaction of the 40S subunit with domain II is restricted to its apical half, and consistently, substitutions in domain II that affect IRES function most severely are localized to this region [32,68,69]. The absence of domain II impairs 48S complex formation [48]. Recent studies suggest that it is also absolutely required for 80S complex formation [31], although this conclusion is contradicted by the ∼20% residual activity of mutant IRESs that lack this domain (e.g., [31,32]). Nevertheless, possible involvement of domain II in a late stage of initiation could be a consequence of the conformational changes that it induces in the 40S subunit.
Although there are striking similarities between initiation in prokaryotes and initiation on HCV-like IRESs, such as factor-independent binding of ribosomes to mRNA, and inhibition of initiation by sequestration of the initiation codon in even relatively weak secondary structure (cf. [10], and see a discussion in [33]), there are also significant differences. Whereas ribosomal binding in prokaryotes is primarily determined by base-pairing of the linear SD and anti-SD sequences, it is evident that 40S subunits bind to multiple non-contiguous determinants on HCV-like IRESs, and that binding is a multi-step process that involves induced conformational changes in the 40S subunit and probably also in the IRES. It is likely that further conformational changes occur in the IRES during subsequent steps in the translation process. For example, domain II likely remains bound to the E site after subunit joining [70] but must be displaced for elongation to proceed.
Specific contacts between the IRES and components of the 40S subunit have not yet been at high resolution. It is not known whether the IRES binds specifically to 18S rRNA, but it can be cross-linked to several ribosomal proteins [48,71,72]. By comparison with homologous components of the 30S subunit, they map to the protein-rich solvent-exposed side of the 40S subunit, consistent with the site of binding revealed by cryo-EM [60].
2.4 HCV-like IRESs in animal and avian picornaviruses
Initiation on the HCV IRES differs fundamentally from the canonical mechanism of initiation in terms of the mechanisms used for ribosomal attachment to mRNA and to locate the initiation codon. As only three different mechanisms of internal ribosomal entry are known to date (epitomized by EMCV, HCV and CrPV IRESs), a fundamental question is whether other IRESs have evolved to use additional distinct initiation mechanisms, or whether newly identified IRESs will be found to be structurally and functionally related to the three known groups. IRESs that use an HCV-like mechanism were initially identified only in related members of the family Flaviviridae, such as GBV-B and various pestiviruses but recent observations indicate that the mechanism is more widespread. Several groups of animal and avian picornaviruses contain 5′UTRs that are closely related to HCV and particularly to pestivirus IRESs (∼50% overall identity) and that have the potential to adopt a fold that closely resembles that of those IRESs (Fig. 2). The 5′UTR of porcine teschovirus type 1 (PTV-1) has been shown to contain an IRES, and its borders match the structure proposed here [73,74]. The proteins encoded in the open reading frame immediately downstream of the confirmed or putative IRESs in these picornavirus genomes are apparently unrelated. There are significant sequence similarities between these 5′UTRs and the HCV-like IRES in domain III, particularly in the pseudoknot and the functionally important IIId and IIIe hairpins. Apical loop sequences in these domains are nearly completely identical in these picornavirus IRESs and in HCV, pestiviruses and GBV-B. Homology between the predicted structures of each of these groups of picornavirus 5′UTRs is high, so that substitutions in different strains and isolates are either covariant (thereby maintaining a common structure) or occur in single-stranded regions (so that base-pairing is not affected) (our unpublished data). There are significant differences between these IRESs, for example, in the apical IIIabc region of domain III, which ranges in size from ∼80 nt. in PTV-1, to 110 nt. in HCV and PEV-8 and up to 125 nt. in simian picornaviruses. Despite this variation, the basic mechanism of initiation on all of these IRESs is likely identical: just as for HCV, the PTV-1 IRES binds directly to 40S subunits in the absence of all initiation factors, binds to eIF3, and requires only 40S subunits and the eIF2 ternary complex to form a 48S complex on the initiation codon [74].

Proposed structures of IRES domain IIId, domain IIIe and the pseudoknot of (A) avian encephalitis virus (Calnek vaccine strain: AJ225173), (B) porcine enterovirus type 8 (PEV-8: AF406813), (C) duck picornavirus TW90A (AY563023), (D) porcine teschovirus (PTV) type 1 (AF231769) and (E) simian picornavirus (SPV) type 9 (AY064717). BLAST (http://www.ncbi.nlm.nih.gov/BLAST) was used to identify sequences on the basis of homology with conserved elements of these domains in HCV and pestivirus IRESs; folding was done using MFOLD [75] to determine secondary structures and PFOLD [76] to verify these structures and for identification and folding of the pseudoknot. The PTV-1 structure is valid for all eleven PTV serotypes and the SPV-9 structure is valid for SPV types 1, 3, 4, 9, 11, 12, 13 and 15. The AEV structure is also valid for SZ and van Roekel strains (AY275539 and AY517471, respectively). The PEV-8 structure is valid for all known strains (AY392556, AY392545, AY392544, AY392543, and AY392538). Sequence variation within groups is limited, but favors the proposed structures.
The polyproteins encoded by the picornaviruses shown in Fig. 2 are typical of the Picornaviridae, except for the coding sequences immediately adjacent to the IRES. However, the sequence and structure of these 5′UTRs are unrelated to the major groups of picornavirus IRESs, but resemble those of the IRESs of the Flaviviridae BVDV, CSFV, and HCV. It appears likely that these picornaviruses acquired HCV-like IRESs by non-homologous recombination, possibly with a cellular mRNA, but more likely with a viral genome that contained a flavivirus-like IRES. There is a precedent for such a possibility: the genomes of GB viruses A and C encode polyproteins that are identical in gene order and distantly related by sequence to HCV, but instead of an HCV-like IRES and the adjacent N-terminal core protein gene, contain functional IRESs that have some picornavirus-like characteristics and appear unrelated to the HCV IRES [77]. The identification of HCV-like IRESs in a distinct family of viruses indicates that this mechanism of initiation is more widespread than anticipated, and leaves open the possibility that cellular mRNAs may exist that contain similar IRESs.
3 Initiation of translation without the involvement of initiator factors or initiator tRNA
3.1 The structure of the intergenic region (IGR) IRESs of dicistroviruses
The genomes of the dicistrovirus family of positive-sense RNA viruses contain two large open reading frames (ORFs) that encode nonstructural and capsid proteins, respectively (Fig. 3A). They are separated by intergenic regions of ∼190–220 nt. that have been proved to be IRESs in Plautia stali intestine virus (PSIV), cricket paralysis virus (CrPV), Rhopalosiphum padi virus (RhPV) and Taura shrimp virus (TSV) [78–82]. A profound shut-off of host cell protein synthesis occurs following infection of cells by dicistroviruses (e.g., [83]), but this does not affect synthesis of viral capsid proteins, which are synthesized in great excess over nonstructural proteins from about 3 h post-infection [84].
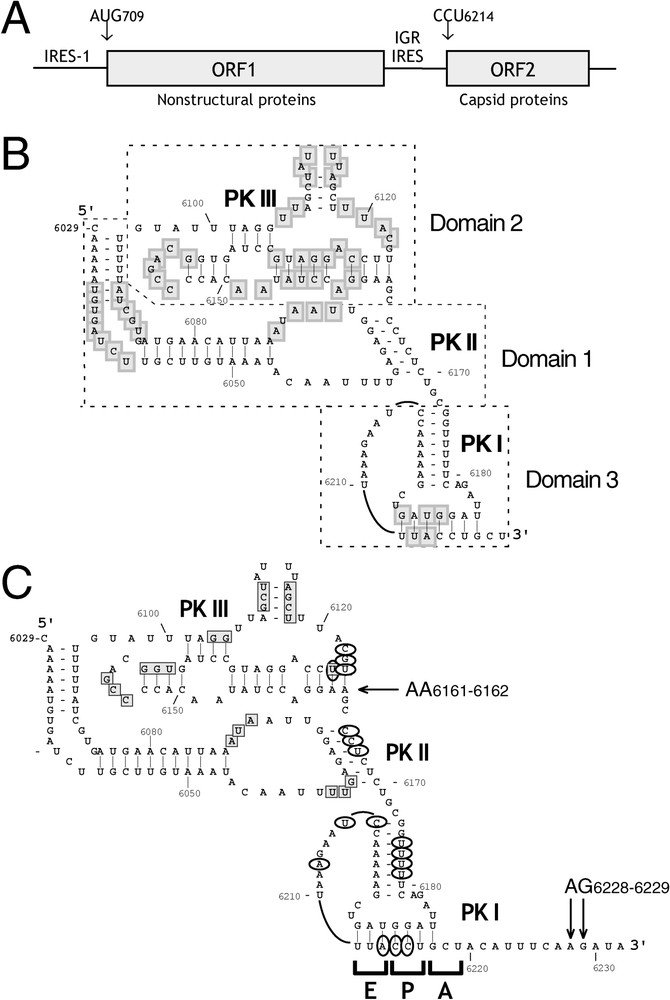
(A) A diagrammatic representation of the structure of the CrPV genome, showing the intergenic region (IGR) IRES between open reading frames that encode nonstructural and capsid proteins. (B, C) Secondary structure models of the CrPV IGR IRES [85,86]. (B) Model of the IRES annotated to show domains 1, 2 and 3, and pseudoknots I, II and III (as indicated), and those nucleotides (shaded gray) that are conserved in at least seven of the eight CrPV-like IRESs (Aphid lethal paralysis virus (AF536531), Black queen cell Virus (AF183905), Cricket paralysis virus (AF218039), Drosophila C virus (AF014388), Himetobi P virus (AB017037), Plautia stali intestine virus (AB006531), Rhopalosiphum padi virus (AF022937), and Triatoma virus (AF178440)). (C) Model of the IRES annotated to show toeprints (black arrows) due to binding of 40S subunits and 80S ribosomes [70,93], sites on CrPV and PSIV IRESs (light grey squares) protected from chemical and enzymatic cleavage by bound 40S subunits [85,88] and sites of cleavage (circles) induced by Fe(II)-eIF1 derivatives in complexes with the CrPV IRES and 40S subunits [63].
Chemical/enzymatic probing and mutational analyses of CrPV, PSIV and RhPV IRESs [79,85,86] and phylogenetic analysis of the 12 currently known IGR sequences [80,87] indicate that they have closely related tertiary structures, consisting of three domains, each of which contains a pseudoknot (PK). PKII and PKIII overlap and likely form a stable folded domain, whereas PKI is attached to them by a linker and is less rigid [85,86]. IGR IRESs can be divided into two groups. The predicted structures of 8 IGR IRESs resemble that of the CrPV IRES (Fig. 3). However, the IGR IRESs of Kashmir Bee Virus (KBV), acute bee paralysis virus, Solenopsis invicta virus 1 and Taura shrimp virus likely have a slightly different arrangement of helices within domain 2 and contain an extra hairpin in PKII. Mutational analyses have confirmed the importance of the structure of IGR IRESs for their function: disruption of secondary structure elements led to loss of activity, which was partially or wholly restored by compensatory second-site suppressor mutations that restore base-pairing [79,80,82,86,88]. Some conserved nucleotides in the CrPV subgroup of IRESs are not present in the KBV subgroup, whereas other unpaired sequences are conserved in both subgroups. This conservation, and the loss of IRES function caused by mutation of conserved, unpaired regions [85,86,88] suggests that sequences that are conserved only in the CrPV subgroup play a structural role in determining the fold of the IRES, whereas elements present in both subgroups may play specific functional roles.
A remarkable property of these IRESs is that the structural protein gene lacks an AUG initiation codon; instead, depending on the virus, initiation occurs at GCU, GCA or GCC codons encoding alanine or at a CAA codon encoding glutamine at the 3′-border of the IRES [55,79,81,82,89]. There is no absolute requirement for any of these triplets, and initiation occurred at precisely the same site whatever coding triplet was present there [70,90,91]. Differences in the utilization of the alternate start codons are likely due to attendant minor changes in local secondary structure that impair binding of the cognate region of the IRES to the ribosomal decoding region. Translation is optimal when the coding region immediately adjacent to the start codon is single-stranded [91]; retention of ∼50 nt. of the viral sequence upstream of the PSIV IRES enhances translation of heterologous coding sequences [92], presumably by “buffering” the IRES against potential induced structural changes. Analysis of translation products indicated that methionine is not the initiating amino acid for translation mediated by PSIV, CrPV and RhPV IRESs [79,82,89] and reconstitution of the translation process in vitro over several elongation cycles confirmed that this process does not involve Met-tRNAMeti [70]. Translation from the CrPV IRES is inhibited by addition of eIF2 to in vitro translation extracts and is enhanced by reductions in the level of active eIF2-GTP/Met-tRNAMeti ternary complex in cells [93,94]. The implication that the IGR IRES and the eIF2 ternary complex compete for binding to a common site on the 40S subunit has been confirmed directly (see below).
3.2 The mechanism of initiation on the dicistrovirus IGR IRES
Prior to identification of IGR IRESs, a key aspect of those mechanisms of IRES-mediated initiation that had been elucidated was that they depend on specific non-canonical interactions of the IRES with canonical components of the initiation apparatus [95]. These interactions are specific, so that, for example, the EMCV IRES binds to mammalian but not wheat eIF4G or eIF(iso)4G [48], and the HCV IRES binds to mammalian but not wheat 40S subunits [48]. IGR IRESs are exceptional because they are active in every eukaryote tested to date, including insects, mammals, plants and yeast [82,94,96].
Systematic omission experiments showed that the CrPV IRES binds specifically to 40S subunits in the absence of initiation factors, and toeprinting assays showed that this leads to a base-paired CCU triplet in pseudoknot III being placed in the P site, mimicking the base-paired AUG codon/Met-tRNAMeti anticodon of conventional 48S complexes (Fig. 4; [93]). The adjacent GCU codon, which is the first to be translated, is placed in the A site. A 60S subunit can then join this 40S subunit/IRES complex to form an 80S ribosome [93]. Alternatively, the IRES is also able to bind directly to preassembled 80S ribosomes to yield functional initiation complexes that are competent to begin elongation [63]. IGR IRESs do not bind to 60S subunits [88], which implies that the 40S subunit contains the major determinants of the IRES' interaction with 80S ribosomes. The binding site on the 40S subunit is likely to consist of highly conserved elements, because IGR IRESs can also bind to purified insect ribosomes in a factor-independent manner [88]. The ability of the IGR IRES to bind to 80S ribosomes is unprecedented in a eukaryotic mRNA. It may favor selective translation of dicistrovirus capsid proteins in infected insect cells and thus contribute to the rapid replication cycle of dicistroviruses if, by analogy with animal cells infected with picornaviruses such as poliovirus and mengovirus, shut-off of host cell protein synthesis is initially accompanied by polysome disaggregation and 80S monosome accumulation [29,97,98].
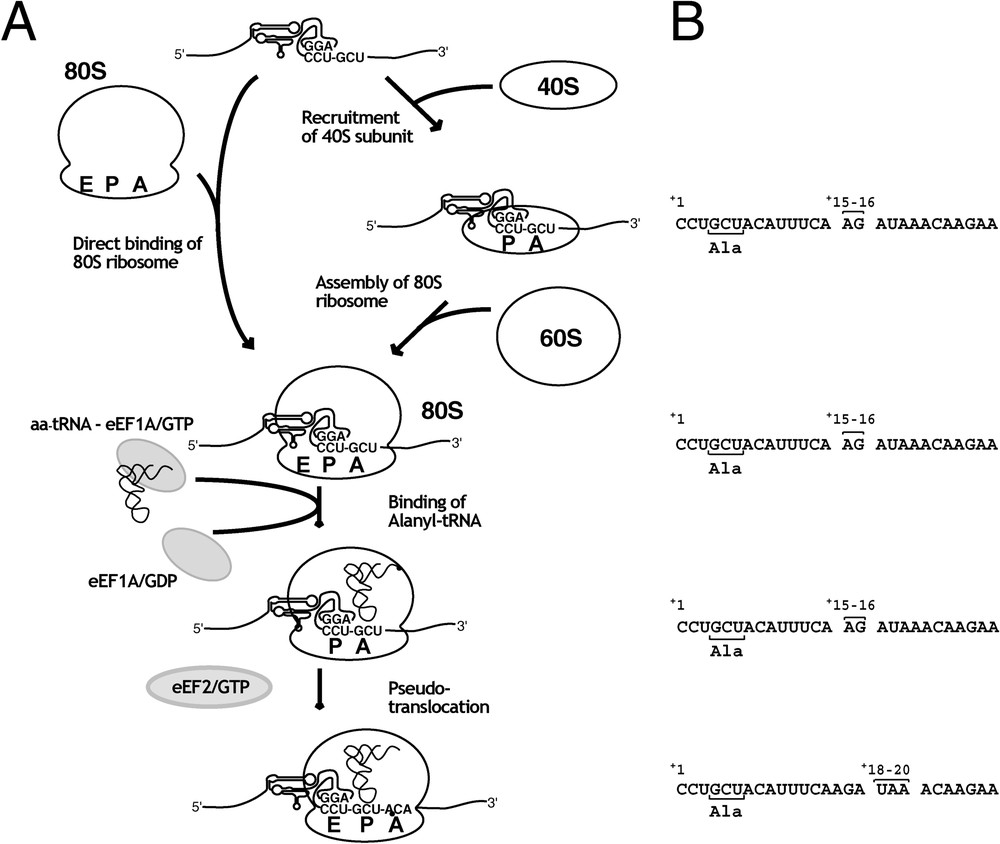
Model for the mechanism of translation initiation on the CrPV IGR IRES. (A) Assembly of ribosomes on the IGR IRES can occur either by direct binding of 80S ribosomes to the IRES or by factor-independent binding of the 40S subunit, followed by joining of the 60S subunit to the IRES/40S subunit complex. The usual interaction between the anticodon of Met-tRNAMeti and the P site AUG initiation codon is substituted by base-pairing between the CCU P-site triplet and upstream sequences, which are both part of pseudoknot I. Ala-tRNAAla is delivered to the A site by EF1 and base-pairs with a cognate GCU codon. Subsequently, EF2 mediates a ‘pseudo-translocation’ event (in the absence of peptide bond formation) placing the base-paired GCU codon of CrPV mRNA and the AGC anticodon of Ala-tRNAAla in the P-site, and the base-paired CCU:GGA triplets of pseudoknot I in the E site. Subsequent elongation cycles occur in a conventional manner. (B) The position of the leading edge of the ribosome on CrPV mRNA at each step in the pathway as determined by toeprint analyses [63,70] indicated above nucleotides 6214–6240 of the CrPV genome. By convention, +1 refers to the first nucleotide of the triplet in the P site of the initiation complex.
The modes of interaction of the HCV-like and IGR IRESs with the 40S subunit differ significantly. Binary 40S subunit/CrPV IRES complexes yield two sets of toeprints: the position of one, at AA6228–9 (downstream of the first decoded triplet), indicates that the base-paired CCU triplet in PKI occupies the P site. The second, at AG6161–2 at the 3′ edge of domain 2 is indicative of stable interaction of an adjacent region of the IRES with the 40S subunit [93]. Footprinting assays revealed that the surface of PSIV and CrPV IRESs that is most stably bound to the 40S subunit is limited to domains 1 and 2 [85,88]; Fig. 3). Consistent with these observations, surface-exposed nucleotides in the IGR IRES bound to the 40S subunit barely overlapped the IRES residues that are protected by the bound 40S subunit [63]. Exposed nucleotides were identified by directed hydroxyl radical probing, using [Fe(II)-BABE]-derivatized eIF1 mutants bound to 40S subunits as probes. Mutations in PKI that abrogate IRES function caused minimal reductions in the affinity of binding to the IRES and did not prevent the stable interaction of the 40S subunit with domain 2 of the IRES, whereas mutations in PKII and PKIII impaired binding to a much greater extent [85,88]. These observations indicate that the placement of domain 3 in the ribosomal P site is dependent on an initial higher-affinity interaction of 40S subunits with the upstream domains 1 and 2. This suggested stepwise binding process resembles that proposed for binding of HCV and CSFV IRESs to the 40S subunit, during which entry of mRNA flanking the initiation codon into the mRNA-binding cleft was also dependent on initial high-affinity interactions involving upstream parts of the IRES.
The factor requirements for translation from the CrPV IRES have been determined in detail [63,70]. A systematic examination of the influence of factors on successive stages of the unusual process of initiation on this IRES showed that the eIF2-GTP/Met-tRNAMeti ternary complex alone had no effect, but that combinations of eIF3, eIF1 and eIF1A together with this complex competitively inhibited binding of the IRES to 40S subunits in direct proportion to their ability to promote binding of the ternary complex to 40S subunits [63]. Joining of 60S subunits to IRES/40S subunit complexes was impaired by eIF3 alone, and to a greater extent together with eIF1: these observations are consistent with the ribosomal anti-association activities of these factors [3,4,94,99]. Unexpectedly, this inhibitory effect was not suppressed by eIF5 and eIF5B, which displace eIF3 and eIF1 from the 40S subunit during the subunit joining step on a conventional capped mRNA [5,8]. This failure could be because eIF5B is prevented by the IRES from interacting normally with the 40S subunit by steric hindrance, because of conformational changes induced in the ribosome by the IRES (see below), or because eIF5B acts to displace other factors from the 40S subunit only when aminoacyl-tRNA is present in the P site. eIF1A is a homolog of the prokaryotic initiation factor IF1, which binds to the A site [100]. The possibility that eIF1A binds to the equivalent site on eukaryotic ribosomes, blocking access of elongation factor 1 (EF1)-GTP/tRNA, could explain why this factor inhibits elongation following ribosomal binding to the IRES.
Direct binding of the IRES in such a way that initiator tRNA is excluded from the P site and such that the first decoded triplet is initially situated in the A site suggests a model in which translation begins after aminoacyl-tRNA has been brought to the A site of the 80S ribosome, presumably by EF1, and has been translocated to the P site. This second step could theoretically be mediated by EF2 or be induced by the bound IRES itself. The first elongation step would be exceptional in either scenario, because it occurs without aminoacyl tRNA in the P site, and consequently without prior peptide bond formation. Reconstitution of this process on mRNA templates comprising the CrPV IRES and defined heterologous coding sequences together with assays for peptide bond formation confirmed that functional 80S ribosomes did assemble on the IRES in a factor-independent manner, and indicated that they proceed directly to the elongation phase of translation [70,90]. The first and subsequent elongation cycles depended strictly on EF1-mediated delivery to the A site of the aminoacyl-tRNA cognate for each triplet and on EF2, initially for translocation of the initiating aminoacyl-tRNA to the P site, and subsequently for translocation of peptidyl-tRNA.
The deduced mechanism of initiation implies that the CrPV IRES must play several distinct roles in this process. These include binding specifically and with high affinity to the 40S subunit, mimicking the base-paired AUG codon and Met-tRNAMeti anticodon in the P site, setting the correct reading frame for translation accurately, and promoting the initial translocation event. Understanding how the IRES carries out these functions will require a detailed understanding of how these different elements interact with the ribosome. Cryo-EM structures of the IRES bound to 40S subunits and to 80S ribosomes (at a resolution of ∼17–20 Å) show that it binds in the intersubunit space, overlapping the binding site of E site tRNA and extending through the P site to the A site [101]. These data and the results of directed hydroxyl radical probing [63] are consistent. These interactions are altered slightly following subunit joining. In the 80S ribosome, the IRES interacts directly with ribosomal proteins S5, L1 and L11, with helix 30 of 18S rRNA and with helices 79 and 84 of 28S rRNA [101], which form parts of conserved binding sites for P and E site tRNAs.
The mode of binding of the CrPV IRES to the 40S subunit is thus very different from that of the HCV IRES [60] although there is some overlap in the region of the E site occupied by the CrPV IRES and domain II of the HCV IRES. As with HCV IRES, binding of the CrPV IRES also induces conformational changes in the 40S subunit, which are surprisingly similar, consisting of clockwise rotation of the head relative to the body, as well as localized changes that lead to rRNA helices 18 and 34 forming a latch structure in the mRNA entry channel that likely clamps the incoming 3′ region of the mRNA. This rotational movement of the head is reversed after subunit joining. The presence of the IRES in the resulting 80S ribosome leads to a conformational ordering of P proteins in the stalk. It has been suggested that this may facilitate the ribosome's direct entry into the elongation phase of translation because of the stalk's activity in binding to and activating GTPase elongation factors [101].
4 Conclusion
Initiation on the HCV-like and CrPV-like classes of IRES described here occurs by processes that involves their specific, factor-independent binding to the 40S subunit. Although the mechanisms by which these IRESs mediate initiation have not been fully elucidated, it is apparent that these two classes of IRES bind to the 40S subunit in very different ways. The primary high-affinity interaction of the HCV IRES occurs on the solvent side of the 40S subunit, and is required for placement of the initiation codon in the P site. Initiation requires the eIF2 ternary complex, eIF3 and thus probably both eIF5 and eIF5B. By contrast, the initial high affinity interaction of the CrPV IRES occurs in ribosomal P and E sites, excludes initiator tRNA from the P site, and is required for placement of the first decoded triplet in the A site. Neither initiation factors nor initiator tRNA is required for assembly of functional ribosomes that are competent to begin translation. Nevertheless, there are similarities between these two mechanisms of initiation: it appears that binding of both IRESs is likely to be at least a biphasic process, i.e. initial high-affinity binding, followed by “accommodation” of sequences flanking the start codon in the mRNA-binding channel of the 40S subunit. Binding of both IRESs to the 40S subunit induces substantial conformational changes in it: the possibility that these changes are integrated with the “accommodation” process is clearly an attractive possibility.
In light of the identification of HCV-like IRESs in a broad range of picornaviruses, it is apparent that other mRNAs outside the Flaviviridae can use an HCV-like mechanism for initiation. Moreover, the substantial nucleotide insertions present in some IRESs of this type (such as the doubling in size of GBV-B domain II relative to HCV domain II [19], or the presence of an additional hairpin in CSFV domain IIId) indicate that this initiation mechanism is compatible with significant sequence variability, as long as essential functional motifs are present. These observations indicate that it is highly probably that other mRNAs will be identified that use an HCV-like mechanism for IRES-mediated initiation. A more interesting question is whether novel mechanisms of IRES-mediated initiation by factor-independent binding to ribosomes remain to be identified. Since the first three mechanisms of IRES-mediated initiation to have been examined in detail (i.e. EMCV, HCV and CrPV) all use fundamentally different mechanisms, this must be considered a real possibility.
Acknowledgements
This work was supported by Grant AI51340 from the National Institutes of Health.