1 Introduction
Neural stem cells (NSCs) are the self-renewing, multipotent cells that generate the main phenotypes of the nervous system (Fig. 1). During development, NSCs participate to the formation of the nervous system. It was believed that the generation of neuronal cells in mammals was mostly limited to the pre-natal phase of development, and that the adult brain was devoid of stem cells, and thus of the ability to make new nerve cells and regenerate after injuries [1]. Seminal studies in the 1960s, that were substantiated in the 1970s and 1980s, reported that neurogenesis occurs in discrete areas of the adult brain in rodents [2–6]. With the advent of new methods for labelling dividing cells, such as 5-bromo-2′-deoxyuridine (BrdU, Fig. 1) labelling, retroviral labelling and confocal microscopy, investigators have confirmed that neurogenesis occurs in discrete areas of the rodent brain throughout adulthood [7–12], and reassessed and presented evidences that adult neurogenesis also occurs in primates, humans and nonhumans [13–15]. BrdU is a thymidine analogue used for birth-dating and monitoring cell proliferation [16]. BrdU is generally administered intraperitoneally; it inserts into the DNA of dividing cells, including in the central nervous system (CNS), as it crosses the blood–brain barrier [17]. It is hypothesized that newly generated neuronal cells originate from stem cells in the adult brain. A hypothesis further supported by the recent isolation and characterization of neural progenitor and stem cells from the adult brain [18,19]. The confirmation that neurogenesis occurs in the adult brain and NSCs reside in the adult CNS have profound implications for our understanding of brain development and functioning, as well as for cellular therapy in the CNS.
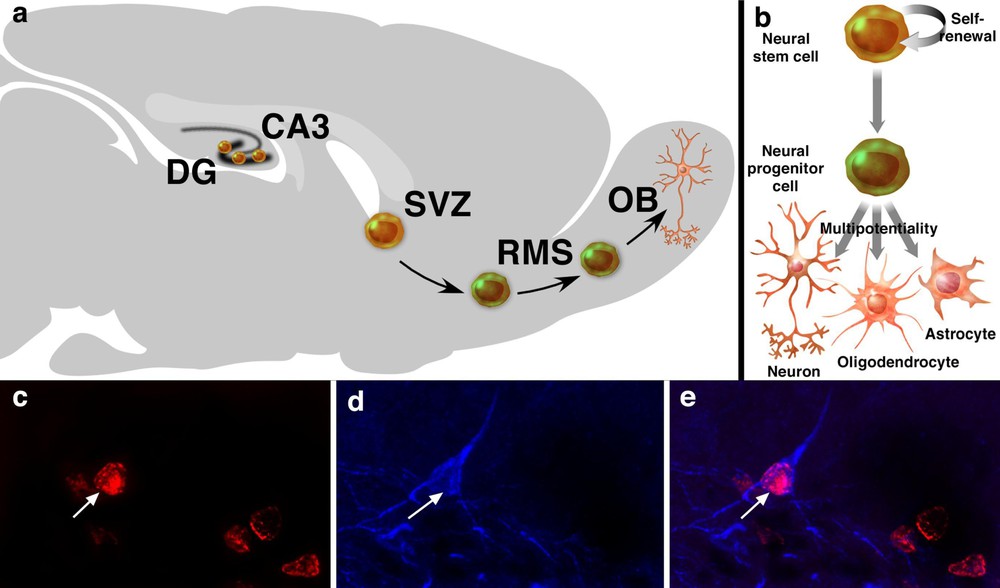
Neurogenesis and neural stem cells in the adult brain. Neurogenesis occurs mainly in two areas of the adult brain, the DG and the SVZ. In the DG, new neuronal cells are generated in the subgranular zone. In the SVZ, newly generated neuronal cells migrate to the OB, through the RMS, where they differentiate into interneurons of the OB (a). NSCs are the self-renewing, multipotent cells that generate the neuronal and glial cells of the nervous system. Neural progenitor cells are multipotent cells with limited proliferative capacity (b). 5-Bromo-2′-deoxyuridine-labelling is the standard for studying neurogenesis and its regulation. Co-labelling of a BrdU-positive cell (c, red, arrow) with class III β-tubulin isotype (d, blue) in the DG. Tuj-1 is a marker of immature neuronal cells. The merge picture shows a BrdU-positive cell also positive for Tuj-1 (arrow, e) in the DG, representative of a newly generated neuronal cell.
2 Neurogenesis in the adult brain
Neurogenesis occurs in two forebrain regions of the adult brain: the subventricular zone (SVZ) and the dentate gyrus (DG) in various species [20]. Of these two regions, the SVZ harbours the largest pool of dividing neuronal progenitor cells in the adult rodent brain [21,22]. Newly generated neuronal cells in the SVZ migrate to the olfactory bulb (OB) through the rostro-migratory stream (RMS), where they differentiate into granule and periglomerular neurons of the OB, in rodents [4,10,23] and in macaque monkeys [15,24] (Fig. 1a). Macaque monkeys are nonhuman Old World anthropoid, phylogenetically close to humans. Like humans they have a hippocampal formation, a relatively small OB – compared with rodents –, a life-history pattern and are largely diurnal [25,26]. Progenitor cells in the SVZ aggregate to form an extensive network of neuroblast chains that migrate tangentially through the SVZ and coalesce anteriorly to form the RMS [27,28]. The network of chains of migrating neuroblasts in the SVZ and RMS can be revealed by immunostaining for polysialylated neural cell adhesion molecule (PSA–NCAM) [15,29]. Neuroblasts undergoing ‘chain migration’ along the SVZ/RMS migrate along one another via homotypic interactions, without radial glial or axonal guides [30]. The directional migration of neuroblasts along the SVZ requires the beating of the ependymal cilia [31]. In rodents (mice), a SVZ progenitor cell requires at least 15 days to be generated, migrate 3–5 mm and differentiate into a new olfactory interneurons. These cells migrate at an average rate of 30 mm h−1 [10]. In macaque monkeys, a SVZ progenitor cell requires at least 75 to 97 days to be generated, migrate 20 mm and differentiate into new olfactory interneurons, a process slower than in rodents [15]. In the DG, newly generated neuronal cells in the subgranular zone (SGZ) migrate to the granule layer, where they differentiate into neuronal cells and extend axonal projections to the CA3 area of the Ammon's horn in rodents [3,5–7,11,32,33], in New-World monkeys (marmoset) [13] and in Old-World monkeys [34,35] (Fig. 1c–e). In rodents (rats), immature granule cells extend axons into CA3 as rapidly as 4–10 days after mitosis [36], whereas the maturation of newborn cells, from the proliferation newly generated cells in the SGZ to the migration and differentiation in neuronal cells of the granule cell layer, takes approximately four weeks [7]. As many as 9000 new neuronal cells are generated per day in the rodent DG, contributing to about 3.3% per month or about 0.1% per day of the granule cell population [37,38], whereas in adult macaque monkey, it is estimated that at least 0.004% of the neuronal population in the granule cell layer are new neurons generated per day [35]. The relative rate of neurogenesis is estimated to be approximately 10 times less in adult macaque monkeys than that reported in the adult rodent DG [35]. However, it is difficult to make a quantitative comparison between the species, as nothing is known of the relative bioavailability of BrdU or its ability to cross the blood–brain barrier and be available for uptake by dividing cells in the two species. Neurogenesis may also occur in other areas of the adult brain – albeit at lower level –, such as the CA1 area [39], the neocortex [40,41], the striatum [42], the amygdala [43], the substantia nigra [44], the third ventricle [45], the subcortical white matter [46], the caudate nucleus [47] in some species [19–25]. However, some of these data have been the sources of debates and controversies and remain to be further confirmed [48–50].
In the human, Eriksson et al. [14] performed immunofluorescence labelling for BrdU and neuronal markers on brain tissue samples obtained post-mortem from cancer patients who had been treated with BrdU to assess the proliferative activity of the tumour cells, and revealed that neurogenesis occurs in the DG in the adults [14]. In the SVZ, Sanai et al. (2004) performed immunofluorescence labelling for the cell cycle marker Ki67 on brain tissue specimens from neurosurgical resections and autopsied brains, and reported that the adult human brain contains a ribbon of astrocytes lining the lateral ventricles, a particular organization not observed in other vertebrates, including nonhuman primates. Some cells in the astrocytes ribbon divide, as revealed by the co-expression of glial acidic fibrillary protein (GFAP) and the cell-division marker Ki-67. However, Sanai et al. found no evidence of chains of migrating neuroblasts in the SVZ or in the pathway to the OB. These results suggest that neurons in the adult human OB are not replaced [51]. Alternatively, precursors migrate as individual cells, or the pathway may be different in the human SVZ and yet to be found. But it highlights the particularity of neurogenesis in the adult human brain. The rate of neurogenesis in the human DG was also reported to be low [14]. The reasons for the apparent reduction of neurogenesis in adult primates are unclear. The decline of adult neurogenesis during vertebrate evolution could be an adaptative strategy to maintain stable neuronal populations throughout life [52]. This hypothesis is consistent with the restriction of adult neurogenesis in the mammalian brain to phylogenetically older structures, the OB and hippocampal formation, and its absence in the more recently evolved neocortex [48,52,53].
Altogether these data confirmed that neurogenesis occurs in the adult mammalian brain, though the particularities of neurogenesis in human remain to be further determined. Newborn granule cells in the DG survive for extended period of time, at least 2 years in humans [14]. Thus, neuronal cells born during adulthood that become integrated into circuits and survive to maturity are very stable, and may permanently replace granule cells born during development. It is postulated that newly generated neuronal cells originate from residual stem cells in the adult brain [20,54].
3 Neural progenitor and stem cells form the adult brain
In the adult brain, it is hypothesized that NSCs reside in the adult SVZ and hippocampus. In 1992, Reynolds and Weiss were the first to isolate and characterize a population of undifferentiated cells or neural progenitor cells, positive for the neural progenitor and stem cell marker nestin [55], from the adult mice striatal area, including the SVZ [18]. In 1995, Gage et al. isolated a similar population of cells, from the adult rat hippocampus [19]. One of the limitations with the established cultures derived from the adult brain is that they are heterogeneous; they contain neural progenitor and stem cells. Neural progenitor cells are defined as multipotent cells, with limited proliferative capacity [20,56,57] (Fig. 1b). Further, there is no marker of NSCs in vitro and in vivo; the intermediate filament nestin, a marker for neural progenitor and stem cells, is also expressed in vivo in reactive astrocytes and gliomas [58,59]. Other markers of neural progenitor and stem cells, such as the transcription factors sox-2, oct-3/4, and the RNA binding protein Musashi 1, also label population of glial cells [60–64]. Despite intense effort, NSCs are yet to be identified [65–67]. Hence, the characterization in vitro of cells with NSC properties requires demonstrate self-renewal over an extended period of time (more than five passages), multipotentiality, coincident with the generation of a large number of progeny, several orders of magnitude more numerous than the starting population [68,69]. In 1996, Gritti et al. isolated self-renewal, multipotent NSCs from cultured derived from the SVZ, and in 1997, Palmer et al. isolated and characterized self renewal, multipotent NSCs derived from the adult hippocampus [70,71]. Recent data have challenged the existence of NSCs in the adult hippocampus, reporting the isolation of neural progenitor, but not stem cells from the adult hippocampus [72,73]. However, differences in protocols, species, handling technique may explain discrepancies between the studies, and such claim that self-renewal, multipotent NSCs cannot be isolated from the adult hippocampus would require comparing the two models in a strict experimental approach. Altogether, these data suggest that NSCs reside in the adult SVZ and hippocampus. Neural progenitor and stem cells have also been isolated and characterized in vitro form other areas of the adult brain and the spinal cord, suggesting that neural progenitor and stem cells may also reside in other areas of the CNS, where they would be quiescent [20]. The isolation and characterization of neural progenitor and stem cells from the adult brain provide a source of tissue for cellular therapy.
4 Neural progenitor and stem cells in the adult brain
Despite the lack of molecular markers for NSCs, researchers have aimed at identifying the origin of newly generated neuronal cells in the adult brain. Based on ultrastructure, cell cycle analysis, [3H]-thymidine autoradiography and immunocytochemical studies, particularly BrdU-labelling, two conflicting theories with regard to the origin of newly generated neuronal cells in the adult brain have been proposed. One theory contends that newly generated neuronal cells originate from astrocyte-like cells, expressing the glial marker, GFAP, and nestin, in the SVZ [74]. This finding was surprising, because astrocytes are considered as differentiated cells belonging to the glial lineage. The second theory contends that newly generated neuronal cells originate from a population of ependymal cells in the SVZ that express the intermediate filament protein nestin [75]. The ependyma, as the subependyma, originate from the embryonic forebrain germinal zones. However, this conclusion is not supported by other studies [76,77], and remains to be further confirmed [20]. In the hippocampus, Seri et al. [78] and Fillippov et al. [79], using similar approaches, retroviral labelling, studies in transgenic mice expressing the green fluorescent protein (GFP) under the nestin promoter and electrophysiology, showed that SGZ astrocytes are the primary precursors in the formation of new neurons in the adult hippocampus [78,79].
Three main cell types relevant to adult neurogenesis in the SVZ have been identified and characterized in the SVZ, in rodents [80,81] and in macaques [15]. Type-A cells are elongated and smooth cells that do not express GFAP and the intermediate filament vimentine. Type-A cells are immunopositive for nestin, PSA-NCAM-, and β-tubulin (Tuj1). Type-A cells course tangentially to the walls of the lateral ventricle throughout the SVZ [28]. They incorporate [3H]-thymidine, and form chains of migrating cells [27]. Type-A cells correspond to the migrating neuronal precursors or neuroblasts. Type-B cells have irregular shapes. They express nestin, GFAP and vimentine, but are immunonegative for PSA-NCAM and Tuj1. Type-B cells are detected in the SVZ, where they form a tubular trabecula that ensheathes the chains of type-A cells, isolating them from surrounding parenchyma [27]. Type-B cells are astrocytes, and may correspond to the stem cells in vivo. Type-B cells would correspond to the population of relative quiescent cells previously proposed as NSCs in the SVZ [82]. Unlike in the RMS, two types of B cells are distinguished in the SVZ: type B1 and B2. Type-B1 astrocytes separate the chains of type-A cells from the ependymal layer, whereas the basally located type-B2 cells separate the chains of type-A cells from the surrounding striatal parenchyma. Type-B2 (but not B1) cells incorporated [3H]-thymidine. Type-C cells are of large size, have a smooth contour, express nestin, and are immunonegative for GFAP, vimentine, PSA-NCAM and Tuj1. Type-C cells are present throughout the lateral wall of the SVZ, but not in the RMS, where they are often found in clusters, closely associated to the migrating neuroblasts or chains of type-A cells. Type-C cells are the most actively proliferating cells in the SVZ (50% of 3[H]-thymidine-labelled cells were reported as type-C cells). Type-C cells may generate both neurons and glia, and correspond to precursors of the type-A cells, the migrating neuroblasts. From these data, Doetsch et al. [80] proposed a model for neurogenesis in adult the SVZ: type B cells that are NSCs, give rise to neural precursors type C cells in the adult SVZ, that in turn generate type-A cells that migrate in chains through glial tubes formed by SVZ astrocytes (type-B cells) along the RMS, and will differentiate into interneurons in the OB [80]. This model has been confirmed after studying the order of reappearance of different SVZ cell types and their proliferation, after antimitotic treatment with cytosine-β-d-arabinofuranoside (Ara-C). After Ara-C treatment, migrating neuroblasts (type-A cells), and type-C cells are eliminated, but some astrocytes, type-B cells and ependymal cells remain. The SVZ network then rapidly regenerates: first, astrocytes type-B cells divide, two days later, type-C cells reappear, followed at 4.5 days by migrating neuroblasts. By 10 days, the SVZ network is fully regenerated, as in normal mice. These data suggest that Ara-C treatment kills neuronal precursors cells, type-A and -C cells that are actively dividing cells, but spare the NSCs, type-B cells, that were able to regenerate [83].
In the hippocampus, Seri et al. [78] described a population of small electron-dense cells in the SGZ, type-D cells that are of small size, immunonegative for GFAP and are dividing. Type-D cells probably function as transient precursors in the formation of new neurons, as anti-mitotic treatment resulted in the elimination of D cells from the SGZ. It is proposed that SGZ astrocytes, putative NSCs, would give rise to type-D cells, which in turn would give rise to newly generated neuronal cells in the granule cell layer [78]. Type-D cells in the SGZ do not divide as frequently as type-C cells in the SGZ, suggesting that the amplification for neuronal production by transient precursors in the SGZ is probably limited [78,84]. Filippov et al. [79] further characterized the nestin positive cells in the adult hippocampus in nestin-GFP transgenic mice [79]. Filippov et al. described two subpopulations of nestin-GFP-positive cells. Type-1 cells have long processes, with vascular end feet, express GFAP, and have electrophysiological features of astrocytes – cells with delayed-rectifying potassium currents. Type-1 cells correspond to differentiated astrocytes, presumably corresponding to the type-B cells as described by Seri et al. [78]. Type-2 cells are small in size, express GFAP, are immunonegative for S100β, a marker for astrocytes [85], and have electrophysiological features of the earliest steps of neuronal differentiation – cells with sodium currents. Type-2 cells lacking astrocytic features would correspond to the D cells described by Seri et al. [78]. These data further support the hypothesis that NSCs in the hippocampus correspond to a sub-population of astrocytes, and that nestin expressing cells are heterogeneous in the adult brain. These results also indicate that GFAP, an intermediate neurofilament, is also expressed by cell with early neuronal features in the adult DG. Therefore, a population of progenitor cells expresses GFAP, whether or not this alone qualifies them as glial cells or astrocytes.
Altogether, these data suggest that a population of astrocyte-like cells correspond to NSCs in the adult SVZ and hippocampus. Though the a glial origin for newly generated neuronal cells in the adult brain has received much support recently [86–88], the origin and identity of newly generated neuronal cells in the adult brain is still the subject to debates and controversies, and remains to be further evaluated [20]. Song et al. [89] recently reported that astrocytes in the adult brain promote neurogenesis [89]. The proliferation of astrocytes following Ara-C treatment could stimulate the proliferation of a yet unidentified NSC that is slowly dividing and/or is resistant to Ara-C treatment. It also remains to determine unambiguously the relationship between the precursors of newly generated neuronal cells in vivo, and the neural progenitor and stem cells derived from the adult brain and cultured in vitro. The identification of neural progenitor and stem cells in the adult brain suggests the adult brain has the potential for self-repair.
5 A developmental process
During development, gamma aminobutyric acid (GABA) has been reported to act as an excitatory neurotransmitter on embryonic neuronal progenitor cells, whereas in the mature CNS, GABA acts as an inhibitory neurotransmitter [90,91]. Also, during development, the establishment of synaptic connections follows a defined sequence; the GABAergic synapses are formed prior to glutamatergic ones [92]. In the adult brain, in the hippocampus, newly generated neuronal cells in the DG receive GABAergic innervations soon after their migration is completed [93]. Recent data show that the GABAergic synaptic input on neural progenitor cells in the SVZ and on nestin expressing cells – type-2 nestin-positive cells in nestin-GFP transgenic mice – in the adult DG strongly depolarizes their membranes, thereby providing an excitatory input on newly generated neuronal cells [94,95]. The origin and mechanism of the GABA innervation on newly generated neuronal cells in the adult brain remain to be determined. The depolarization of the membrane potential by GABA during development originates from an increased chloride levels in the embryonic neuronal progenitor cells [96]. A similar mechanism could underlie the activity of GABA on newly generated neuronal cells in the adult brain.
During development, the depolarization of embryonic neuronal progenitor cells by GABA plays a role in the differentiation of granule cells [97]. In the adult brain, the GABAergic depolarization of newly generated neural progenitor cells triggers an increase of the concentration of intracellular calcium, through the activation of voltage-gated calcium channels, and the expression of NeuroD [98]. Since NeuroD is a transcription factor that is required for neuronal phenotype generation in hippocampal dentate granule neurons, the GABA depolarization on adult neuronal progenitor cells may also play a role in the neuronal differentiation of adult progenitor cells [99]. It is further proposed that before receiving any synaptic innervations, newborn neurons may sense neuronal network activity through local ambient GABA levels [100]. Because many physiological and pathological stimulations, such as neurosteroids and epilepsy, affect GABA signalling [101–103], such mechanisms may influence the integration of new neurons in the adult brain.
Therefore, adult neurogenesis may reproduce developmental processes to integrate newly generated neuronal cells in the hippocampal network. GABA may also exert a trophic activity on neural progenitor and stem cells [104]. In the postnatal SVZ, GABA released by neuroblasts inhibits the proliferation of GFAP-progenitor cells [105]. In the SVZ and RMS, GABA released by astrocyte-like cells control the migration of neuronal precursors [106]. Therefore, GABA may act as a paracrine factor to regulate the proliferation and migration of neural progenitor and stem cells in the adult brain.
6 Redefining CNS physiopathology
A wide range of environmental stimuli, and physiopathological conditions in rodents modulate neurogenesis (Table 1). Environmental enrichment, exercise and learning and memory tasks stimulate neurogenesis, whereas neurogenesis decreases with aging in the DG [11,37,107–109]. Neurogenesis is stimulated in the DG and SVZ, in the diseased brain and after CNS injuries, such as in epilepsy, strokes, traumatic brain injuries, Huntington's and Alzheimer's diseases, and decreases after stress [110–116]. New neuronal cells are also generated at the sites of degeneration where they replace some of the degenerated nerve cells, after experimental strokes [117,118]. Cell-tracking studies revealed that new neuronal cells at the sites of degeneration originate from the SVZ. They migrate to the sites of degeneration, partially through the RMS [117,118]. In most of these studies, neurogenesis was quantified by BrdU-labelling. Recently, Kuan et al. [119] provided evidences that hypoxia-ischemia triggers neurons to re-enter the cell cycle and resume apoptosis-associated DNA synthesis in the brain, without cell proliferation [119]. This report cautions the use of BrdU-labelling as a standard for studying neurogenesis and its regulation, and highlights the need to not only characterize BrdU-uptake, but also to show evidence of the absence of apoptotic markers, by terminal deoxynucleotidyl transferase-mediated biotinylated dUTP nick end-labelling (TUNEL) method, for example. The modulation of neurogenesis in the DG and OB in response to environmental enrichment, physiopathological conditions suggest the implication of these structures in these processes. Newly generated neuronal cells survive for extended period of time in the adult brain [14], hence environmental enrichment, physiopathological conditions may contribute to reshaping the adult CNS. Whether constitutive and induced neurogenesis recruit specific populations of progenitor cells remains to be elucidated. The ability of the CNS to regulate the generation of new neuronal cells may be use to promote brain repair in the diseased brain, and after CNS injury [120].
Modulation of neurogenesis in the adult brain
Stimuli | Area | Modulation | References |
Environmental enrichment | DG | increase | [37] |
Exercise | DG | increase | [107,109] |
Learning and memory tasks | DG | increase | [108] |
Aging | DG | decrease | [11] |
Epilepsy | DG | increase | [110] |
Stroke | DG | increase | [112] |
Traumatic brain injury | DG | increase | [113] |
Huntington disease | SVZ | increase | [114] |
Alzheimer disease | DG | increase | [115] |
Stress | DG | decrease | [111] |
Investigators have reported that newly generated neuronal cells in the hippocampus are involved in learning and memory, and depression [121–123]. The function of newly generated neuronal cells, particularly in learning and memory, has been challenged by other studies, reporting that increased hippocampal neurogenesis has been observed without improvement of learning and memory performances, that learning enhances the survival of new neurons beyond the time when the hippocampus is required for memory, and that non-specific effects of treatments aiming at inhibiting adult neurogenesis have yet to be ruled out [124–126]. Newly generated neuronal cells may also contribute to tissular homeostasis in the adult brain. The disturbance in the rate of adult neurogenesis would contribute to pathological processes in the CNS, such as in neurodegenerative diseases. However, such hypothesis remains to be investigated. Therefore, newly generated neuronal cells are involved in the physiopathology of the adult CNS, but the function and relative contribution of newly generated neuronal cells to these processes versus the neuronal cells of the pre-existing network remain to be determined. It is estimated that 0.2% of the degenerated nerve cells are replaced in the striatum after middle cerebral artery occlusion, a model of focal ischemia [117]. This low percentage of newly generated neuronal cells at the sites of injury may account for the lack of functional recovery in the injured CNS. The modulation of neurogenesis by environmental stimuli, and in physiopathological conditions may therefore contribute to CNS plasticity, and the generation of newly generated neuronal cells at the sites of injury in the diseased brain and after CNS injuries may represent a regenerative attempt by the CNS [127,128].
7 Cellular therapy in the adult CNS
The confirmation that neurogenesis occurs in the adult brain, and that NSCs reside in the adult CNS suggest that the adult CNS may be amenable to repair. Cell therapeutic intervention may involve the stimulation and transplantation of neural progenitor and stem cells of the adult CNS. Since neural progenitor and stem cells reside throughout the adult CNS, the stimulation of endogenous neural progenitor and stem cells locally would represent a strategy to promote regeneration in the diseased brain and after CNS injury. Particularly, since new neuronal cells that originate from the SVZ, are generated at the sites of degeneration in the diseased brain and after CNS injuries, strategies that promote regeneration and repair may focus on stimulating SVZ neurogenesis. Neural progenitor and stem cells can be isolated from the adult brain, including from human biopsies and post-mortem tissues [129,130], providing valuable sources of tissue for cellular therapy. To support this contention, neural progenitor and stem cells have been grafted in various animals model of CNS diseases and injuries, such as Parkinson's disease and spinal cord injuries, and shown to improve functional recovery [131–137]. In these studies, the release of trophic factors by the grafted neural progenitor and stem cells, and their interaction with the injured brain are believed to play a major role in the recovery process [131–136]. In a recent study where human foetal neural progenitor and stem cells were injected after spinal cord injury in mice, the improvements in walking disappeared following treatment with diphtheria toxin, which kills only human cells – not mouse cells –, suggesting that the cells themselves are responsible for recovery. Administration of stem cells might then not simply stimulate the body to produce some healing factor, but they directly contribute to repair damage themselves [137].
8 Concluding remarks
The confirmation that neurogenesis occurs in the adult brain, and that NSCs reside in the adult CNS, has open a new era in the understanding of the brain development and physiopathology, and for therapy in the adult CNS. Identifying NSCs, the function and contribution of newly generated neuronal cells to the functioning of the CNS are currently the source of intense research, and debates. The isolation and culture of neural progenitor and stem cells from the adult brain provide new opportunities for cellular therapy in the CNS. These studies will contribute to redefine our knowledge of the CNS, and bring NSC research closer to therapy.
Acknowledgements
P.T. is supported by grants from the NMRC, BMRC, and the Juvenile Diabetes Research Foundation.