1 Introduction
Therapies using stem cells are promising approaches for the treatment of neurodegenerative disorders or other brain injuries [1–6]. The clinical use of embryonic or neural stem cells being limited by ethical and scientific considerations, alternative sources of clinically accessible stem cells for cell replacement therapies are under investigation. Among these cells, bone marrow stromal cells (BMSCs) are candidates for therapeutic uses because they can differentiate into many tissue-specific cell phenotypes. These multipotent cells could be induced in vitro to differentiate into osteogenic, chondrogenic and adipogenic cells [7] as well as various other cell types including liver, heart and brain cells [8–11]. Several reports have suggested that they possess in vivo the capacity to differentiate into neuronal-like cells [12–15]. However, the cell fusion hypothesis has also been proposed to explain the observed neuron- or glial-like phenotypes [16–20]. Recently, in a cell mixing coculture of BMSCs and neurons damaged with glutamate or not, Hokari et al. showed that approximatively 20% of the BMSC fused with the neurons within 24 hr after the start of the experiment [21].
In experimental ischemia models, several groups have demonstrated that BMSCs grafts improved neurological scores and recovery of motor functions [15,22–24]. Other groups have found that BMSCs enhanced the proliferation of endogenous neural stem cells, suppressed the death of newly generated cells and increased axonal plasticity [25,26]. In patients with severe cerebral infarcts, it has been shown that autologous BMSCs have beneficial clinical and radiological effects in patients with middle cerebral artery stroke and with severe neurological aftereffects [27].
Molecular mechanisms underlying these effects are not clear but the plasticity of BMSCs is thought to contribute to their efficiency. Recent data suggest that their interactions with the tissue microenvironment via the secretion of soluble factors strongly contribute in tissue repair [28]. BMSCs have been shown to secrete or express growth factors favoring neuron survival and axonal growth when exposed to injured central nervous system tissue [29,30].
In order to verify putative cross-interactions between BMSCs and the nervous tissue, we used GFP-expressing marrow cells (MCs) added to hippocampal organotypic slice cultures. We investigated three main experimental conditions involving: (i) freshly isolated MCs; or (ii) cultivated BMSCs at one passage in vitro; or (iii) BMSCs at five passages. These cells were grafted on hippocampal slice cultures. Our results suggest different in vitro effects of BMSCs used after one or five passages.
2 Materials and methods
2.1 BMSCs harvest and culture
Experiments followed protocols in accordance with the European Union legislation for the care and use of laboratory animals. Mouse bone marrow cells (MCs) were harvested from 4-week-old GFP mice (C57/Bl/6-TgN (β-agt-EGFP)Osb strain [31]) or 4-week-old OF1 mice obtained from local breeder colonies. Femurs and tibias were aseptically excised and the epiphyses and diaphyses were removed. The bone marrow was flushed from the shaft with DMEM (Invitrogen) using a 20-gauge needle. The marrow suspension was disaggregated by pipetting several times and cells were collected by centrifugation (300 g, 10 min). Cells were used without culture (MCs) or after BMSCs separation by attachment to plastic culture dishes. About 20 × 106 bone MCs were plated on a 25 cm2 plastic flask in 10 mL DMEM medium (Invitrogen) complemented with 10% fetal bovine serum (Invitrogen), 100 U/mL penicillin, 100 μg/mL streptomycin and 250 μg/mL amphotericin B (all from Sigma). Cells were incubated at 37 C in a 5% CO2 atmosphere. Twenty-four hours later, non adherent cells were removed [32,33]. The medium was replaced every 3rd or 4th day for about 3 weeks. When confluent, cells were passaged with 0.25% trypsin and 1 mM EDTA (Invitrogen) for 10 min at 37 C and were replated after 1:2 dilution in culture medium. Cells were used after the first passage (BMSC1P) or after the fifth passage (BMSC5P).
2.2 Organotypic hippocampal culture
They were prepared according to the method of interface organotypic culture [34] with modifications [35]. Briefly, the brains from 7–9 days old OF1 mice were removed after decapitation and sliced into 350 μm transverse sections with a McIlwain tissue chopper. Slices were then carefully separated and the hippocampus were dissected in Hanks’ salts solution and transferred onto sterile Millicell membrane (Millicell-CM filters inserts, pore size 0.4 μm, diameter 30 mm, Millipore). Cultures were maintained at 37 C and 5% CO2 in 1 mL of serum-based medium containing 25% Hank's basal salt solution (Invitrogen), 50% Eagle basal medium (Invitrogen), 25% horse serum (Invitrogen) supplemented with 6.5 mg/mL glucose, 1 mM glutamine (Invitrogen), 100 U/mL penicillin, 100 μg/mL streptomycin and 250 μg/mL amphotericin B. The culture medium was replaced twice a week. After 4 days, organotypic hippocampal cultures were used for graft experiments.
2.3 In vitro graft model
MCs, BMSC1P or BMSC5P were deposited directly onto organotypic hippocampal cultures at a density of 10,000 cells per slice in 2 μL BMSCs medium. No additional factors were added to the slice culture medium, which was changed every 72 hrs. Samples were examined for GFP expression by fluorescence microscopy 3, 7 and 10 days after transplantation. MCs, BMSC1P or BMSC5P proliferation was estimated by counting all GFP cells on and around the organotypic hippocampal culture. Control slices were cultivated under the same conditions without adding MCs or BMSCs.
Statistical significance (*p < 0.05, **p < 0.01, ***p < 0.001) between data obtained in treated slices versus non-treated slices was evaluated for each area by using repeated ANOVA with Tukey's posttest. The data are presented as mean ± SEM.
2.4 Immunohistochemistry
Antibodies to neuronal nuclear antigen (NeuN), neurofilament 200 kD (NF), glial fibrillary acidic protein (GFAP) and oligodendrocytic 2,3-cyclic nucleotide 3-phosphodiesterase (CNPase) were used to investigate the phenotype of grafted cells. Cultures were fixed in 1% paraformaldehyde in 0.1 M phosphate buffer (PB) in the dark at room temperature for 2 hrs under gentle agitation. Then, they were washed 3 × 10 min in 0.1 M phosphate buffered saline (PBS) + 0.3% Triton X-100 and incubated overnight with monoclonal antibodies to NeuN (1:500; Chemicon), NF (1:2000; Boehringer), CNPase (1:200; Chemicon) or polyclonal anti-GFAP (1:500; Sigma). Following 3 × 10 min washing, appropriate fluorescent secondary antibodies, either donkey anti-mouse or anti-rabbit conjugated with cyanine 3 (1:2000; Jackson), were applied for 4 hrs at room temperature in the dark. After 3 × 10 min washing, slices were mounted in glycerin and kept in the dark at 4 °C until analysis.
3 Results
3.1 Marrow cells (MCs) experiments
Deposit reproducibility and overall survival/proliferation rates were assessed by GFP-cell counting. Pictures of the same slices were taken 3, 7 and 10 days postdeposit. Slices were outlined and GFP-MCs mapped within and around the tissue borders, as many cells also survived on the membrane around the tissue. After 3 days in culture, 43.9 ± 7.1 (mean ± SEM) GFP-MCs per slice were counted while 10,000 were deposited. This number increased to 70.9 ± 7.9 at day 7 and to 115.1 ± 12.5 GFP-MCs per slice at day 10. This increase could be the result of a proliferation of transplanted cells, but mitotic figures were seldom observed. Many GFP-MCs progressively aggregated (day 7 and day 10) close to the slices’ edges and gradually penetrated them. Cells on the membrane decreased from 118.8 ± 12.24 at day 3 to 94.53 ± 14 at day 7 and 81.13 ± 17.3 at day 10. Thus, the total number of GFP-MCs on the membrane and tissue was fairly constant, showing only a slight increase at day 10 (Fig. 1).
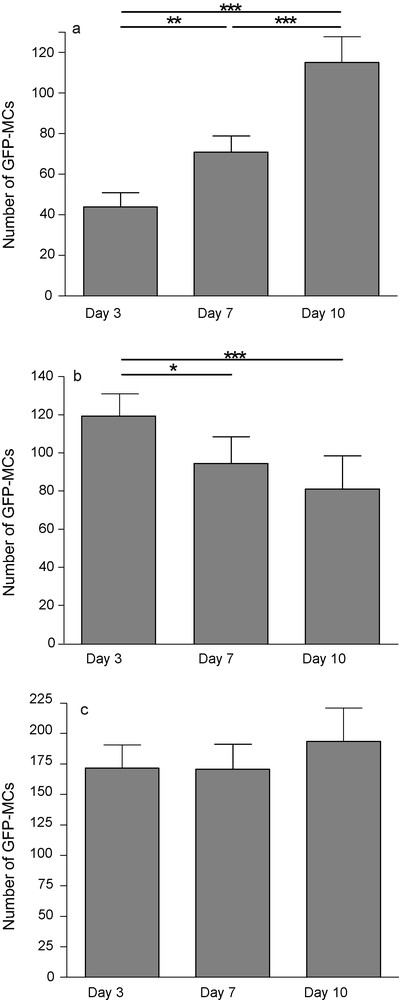
GFP-positive MCs 3, 7 and 10 days after transplantation onto hippocampal organotypic slice culture. The number of fluorescent cells increased on slice (a), decreased on the membrane (b) while the total (slice + membrane) did not change (c). Each value represents the mean ± SEM of 24 samples and was considered significant when *p < 0.05, **p < 0.01, ***p < 0.001; repeated ANOVA measurements with Tukey's posttest.
No long-range migration was observed inside the tissue. Some cells stayed at the same place over 10 days. Others changed orientation or moved a few dozen of micrometers away from their initial position. Finally, some disappeared but cell death was not monitored in this study.
At day 3 postdeposit, most GFP-MCs on slices and membranes were round, about 15 μm diameter (Fig. 2, a). At day 7, many GFP-MC derived cells presented multipolar neural-like morphology (Fig. 2, b). At day 10, the round GFP-MCs were larger than observed at day 3. Some of them displayed two conspicuous nuclear masses (Fig. 2, c).
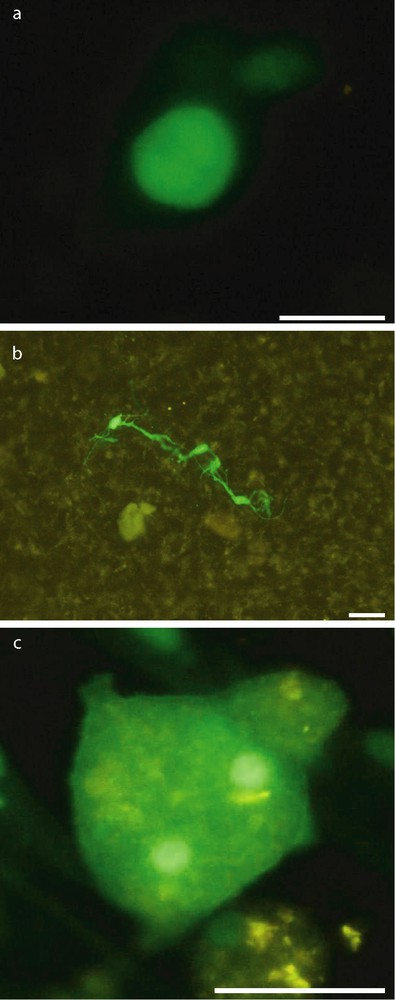
Morphology of GFP-positive MCs after transplantation. Most GFP-MCs on slices and membranes were round 3 days after transplantation (a). Many GFP-MCs derived cells presented multipolar neural-like morphology at day 7 (b). At day 10, some of GFP-MCs derived cells have two conspicuous nuclear masses (c). Scale bar: 30 μm.
GFP-MCs almost never expressed neuronal antigens. Actually, only one on a total of 1700 counted GFP-MCs was stained by NeuN antibody at day 7 (Figs. 3, a and 4). Moreover, this cell did not exhibit neuron-like morphology but was round-shaped. Unexpectedly, some large round-shaped cells contained a granular cytoplasmic labeling compatible with vesicular or lysosomal NeuN location (Fig. 3, b).

GFP-MCs (*) neuronal differentiation. Immunohistochemistry for NeuN. One GFP-MCs derived cell presented the expected nuclear staining for NeuN 7 days after transplantation (a: arrow). The others GFP-MCs presented a cytoplasmic granular labeling of NeuN (b: arrows). Scale bar: 50 μm.

Frequency of transdifferentiated cells, according to their origin. Very few GFP cells expressed NeuN labelling: 1 on a total of 1700 GFP-MCs, 1 on 1500 GFP-BMSC1P and 2 on 1000 GFP-BMSC5P were stained for NeuN.
While very few or no labeling of GFP-MCs in the slices was observed with the NeuN-, NF-, GFAP- or CNPase- antibodies, the corresponding cell types in the slices displayed the expected signals. The staining was more intense in slices grafted with MCs than in control slices at day 3. At day 10, no labeling was observed using NeuN and NF antibodies in control slices, while the grafted slices still exhibited a clear labeling for both antibodies (Fig. 5).

Immunohistochemistry for neuronal proteins (NF and NeuN) of hippocampal organotypic cultures 10 days post MCs graft. (a, b) Immunohistochemistry for NF with (a) or without (b) MCs. (c, d) Immunodetection NeuN with (c) or without (d) MCs. The staining was more intense in slices grafted than in control slices at day 10. Scale bar: 100 μm.
3.2 Bone marrow stromal cells experiments at one passage
After 3 days of culture, 90.79 ± 11.51 GFP-cells were counted over the slices. After 7 and 10 days, this number significantly increased (110.4 ± 15.48 and 115.3 ± 16.89 respectively). On the membranes, the number of fluorescent cells decreased significantly from 228.4 ± 22.65 at day 3 to 175.5 ± 20.93 at day 10, suggesting again a migration from the membrane to the slice that would, at least in part, explain the increased cell counts on the slices. The total number of GFP-BMSC1P remained fairly constant over the 10 days (319.2 ± 28.79; 311.4 ± 31.35; 290.8 ± 33.25) (Fig. 6). No long-range migration was noticed in the slices but some cells moved away from their initial position.

GFP-positive BMSC1P 3, 7 and 10 days after transplantation onto hippocampal organotypic slice culture. Number of fluorescent cells increased on slice (a), decreased on the membrane (b) while the total did not change (c). Each value represents the mean ± SEM of 14 samples. Values were considered significant when *p < 0.05, ***p < 0.001; repeated ANOVA measurements with posttest (Tukey).
At day 3, in the slices, and on the membranes, the GFP-BMSC1P were generally small and round-shaped. At days 7 and 10 on the slices, some GFP-BMSC5P showed processes and a more polygonal appearance; on the membranes they retained their small and round-shaped morphology. As with MCs, the staining (NeuN and NF) was more intense in slices grafted with BMSC1P than in control slices at days 3, 7 and 10 and only 1 on 1500 counted GFP-BMSC1P was stained for NeuN. This cell was round-shaped, as observed for the GFP-MC labeled with NeuN antibody (Figs. 4 and 7).

Immunohistochemistry for neuronal proteins (NeuN) in hippocampal organotypic cultures 7 or 10 days post graft. 10 days after transplantation, the staining for NeuN (a, b) was more intense in grafted slices (b) than in control slices (a). At 7 days, one GFP-BMSC1P derived cell (indicated by arrow) is stained for NeuN (c, NeuN; d, GFP). Scale bar: 500 μm.
3.3 Bone marrow stromal cells experiments at five passages
After three days of culture, 81.25 ± 8.1 were counted on the slices. This represented less than 1% of the 10,000 cells deposited on each slice. After 10 days, GFP-BMSC5P cells survived on and around hippocampal slices. However, their number significantly decreased: 50% of the cells observed at day 3 had disappeared at day 10 (42.8 ± 4). Three, 7 and 10 days after deposit, a similar decrease was also observed on cells that stayed on the membrane (87.63 ± 8.3; 72 ± 7.48; 31.31 ± 2.8), so the total number of cells significantly dropped (168.9 ± 13.35; 134.5 ± 11.28; 74.19 ± 5.77) (Fig. 8).
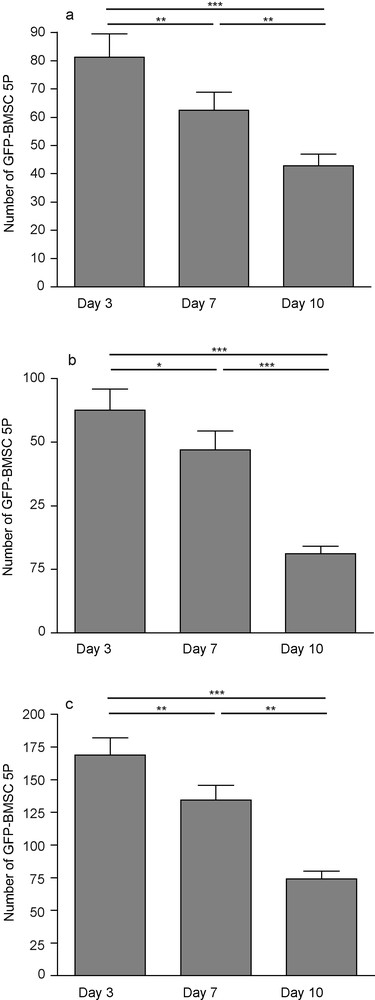
GFP-positive BMSC5P 3, 7 and 10 days after transplantation onto hippocampal organotypic slice culture. The number of fluorescent cells decreased on slices (a) and on membranes (b), so did the total number (c). Each value represents the mean ± SEM of 16 samples and were considered significant when *p < 0.05, **p < 0.01, ***p < 0.001; repeated ANOVA measurements with posttest (Tukey).
The tissue slices have a marked effect on the morphology of GFP-BMSC5P. At day 3 on the slices, they showed round with a small diameter. On the membrane, they were flat and exhibited a very large diameter (about 100 μm × 20 μm). At days 7 and 10, the morphology of the cells on the membrane did not change. On the slices, most cells differentiated long processes and a more polygonal shape at day 7. With time, slice glial cells moved close to GFP-BMSC5P on the membrane and induced GFP-BMSC5P differentiation; these cells then became thinner and differentiated thin processes.
On the membrane as on the slices, GFP-BMSC5P were mobile. To verify if cells migrated preferentially toward the slice, individual or small group of cells were followed. Random changes in orientation, but no clear migration toward or away from the slice were noticed (data not shown).
Very few GFP-BMSC5P derived cells (2‰) expressed NeuN labeling at day 7 (Fig. 4). None were observed at day 3 or day 10. NeuN-GFP cells were round-shaped; they did not display the polygonal shape of other GFP-fluorescent cells. No GFP cell was labeled using NF-, GFAP- or CNPase- antibodies. In the slice cells, no difference could be detected in the intensity or distribution of NeuN, NF, GFAP or CNPase stains in grafted or control slices, suggesting an absence of effect of the BMSC5P on the slices.
4 Discussion
Several studies used hippocampal slice culture model as a powerful means to analyze in vitro interactions between stem cells and differentiated tissues [20,36,37]. In the present study, 10,000 cells in 2 μl medium were layered on slices. This method allowed one to deposit cells all over the slices. The number of GFP-cells on the slices or on the surrounding membranes was assessed after 3 days, immediately before the first medium change. A large majority of the cells had disappeared as only a few dozen actually survived. Thus, less than 1% of the cells deposited stayed on the slices. Then, individual cells or small groups of them could easily be followed during the culture.
The cells that survived on the tissue clearly underwent morphological changes. After three days of culture, MCs, BMSC1P and BMSC5P were small and round-shaped. From day 7, several cell types could be described based on their shape and size. The deposited MCs include multipotent cells, but also their progeny and hematopoietic derived cells. After 10 days on the slices, two dominant MCs derived cell-types were described: large round-shaped cells and medium sized multipolar cells. Some of these cells might express phagocytosis capacities, which could explain the cytoplasmic granular location of the NeuN labeling in some large cells.
The BMSC1P and 5P fraction clearly seemed more homogeneous than the MCs: almost all cells on the slice became multipolar and differentiated multiple processes at day 7 and day 10. However, a few GFP-cells expressed neural markers. This figure probably correlated with the low number of cells on the slices. Nevertheless, we confirmed that BMSCs can differentiate into neuron-like cells, even if this occurred at a very low rate in our conditions.
In vivo and in vitro studies have reported that BMSCs are able to generate cardiomyocytes, hepatocytes or even neural cells as neurons or glial cells [8–15,38–43]. In vitro experiments also demonstrated that these cells differentiated from BMSCs can display neural functional characteristics [44–46]. However, the rate of these differentiations is very low and is not likely to account for the improvement of neurological scores observed in several in vivo experimental models of central nervous system lesions [15,22–24,47].
In the present experiments, the number of passages had a clear effect on the behavior and survival of the grafted BMSCs. While the same number of cells had been deposited, more BMSC1P or BMSC5P were counted after three days of culture on the slices and membrane as compared to the MCs, probably because these cells have a higher capacity to attach to a substrate. After 7 and 10 days of culture, while the number of BMSC5P on the slices and membrane significantly decreased, the total number of MCs or BMSC1P was stable. We observed that BMSC5P became flat and large after 3 days, suggesting that they may enter in senescence [48,49], but we did not verify if some of them engaged in apoptosis or other death program.
The total number of MCs or BMSC1P remained constant, but it increased in the tissue slices and decreased on the membrane, indicating that some cells may migrate toward and in the tissue. Many cells aggregated at the tissue edges, and were then observed within the tissue borders. However, it is worth mentioning that a similar migration of BMSC5P was not noticed.
We also observed that the morphology of the tissue engrafted with MCs was preserved as well as the labeling for neural markers, particularly neurofilament that were readily observed even after 10 days in culture, unlike in control slices. Literature data already described the migration ability of BMSCs, mainly after in vivo lesions [1,14,15,26,50–52]. The neuroprotective action of BMSCs has also been suggested in vivo and in vitro, and therapeutic effects of these cells have been explained by their capacity to enhance endogenous neurogenesis and neurite outgrowth, and to protect newborn cells from deleterious environment [26,29,53–55]. A clinical assay also showed the feasibility, safety and potential efficacy of intravenous injection of autologous MSC in patients with ischemic stroke [27]. Literature data indicate that BMSCs promote tissue repair by secreting soluble factors including brain-derived neurotrophic factor (BDNF), basic fibroblast growth factor (bFGF) and nerve growth factor (NGF) [53,56] more than by true differentiation and cell replacement [57,58]. However, our study points out a clear effect of the number of passages on the BMSCs capacities to migrate in and to protect the hippocampal slice cultures. It has been reported that BMSCs selected after 10 passages may even have deleterious effects on the nervous tissue in an in vitro model that mimics the immediate treatment of stroke with BMSCs secreted factors [59], while protective effect is observed when BMSCs are used after two or three passages [51]. Our observations suggest that BMSC after five passages may enter in senescence and die at a faster rate, which would explain the lack of beneficial effect on the tissue slice.
Our study supports the hypothesis that the effects of cultivated BMSCs on brain slices depend on the number of passages. We also noticed that freshly isolated MCs seem to stimulate neurons and glial cells in the slices. Thus, cell fractions of the MCs may produce growth factors similar to that secreted by cultivated BMSCs. Phenotyping of BMSC1P and BMSC5P and characterization of subpopulations in the BMSC1P has been initiated in order to identify the cells that may offer a potential therapeutic advantage.
Conflict of interest statement
The authors declare that they have no competing financial interests.
Acknowledgements
This work was supported in part with a grant from the “Franche-Comté Alzheimer Association”.