1 Introduction
Plants grown under natural conditions are continuously subjected to various environmental stressors, which are the major constraints to crop production, because they are unpredictable, unavoidable, complex in nature, and change gradually [1–3]. Metal toxicity is one of the major threats to crop production because metals easily enter the food chain, and because most are carcinogens [4]. Among toxic metals, cadmium (Cd) is considered to be of major environmental concern because it ranks seventh among the top-20 toxins and is easily absorbed by plants. Being a highly mobile element, Cd can be easily absorbed by plants, transferred to other parts of the plant, and accumulates in different parts of it [1,5]. Accumulation of Cd in different parts of the plant causes different physiological and biochemical changes and alterations of mineral nutrient uptake and of their metabolism [5,6]. The physiological and biochemical changes due to higher accumulation of Cd in plants are chlorosis, necrosis, leaf rolling, growth inhibition, decreased water potential, and cation efflux; the changes cause alterations in membrane functions, stomatal action, antioxidant metabolism, and activities of several key enzymes; induction of oxidative stress in plant tissues, and even death [1,7,8]. Although Cd is a redox non-active metal, it induces oxidative stress indirectly by increased production of reactive oxygen species (ROS), such as singlet oxygen (1O2), superoxide radical (O2·−), hydrogen peroxide (H2O2), and hydroxyl radicals (OH·), through activating nicotinamide adenine dinucleotide phosphate (NADPH) oxidase [9,10]. The Cd-induced higher production of ROS interacts with the antioxidant defense system [11,12] and disrupts the electron transport chain [13] as well as the metabolism and uptake of essential plant nutrients [6,14]. Moreover, Cd accelerates the generation of cytotoxic methylglyoxal (MG) through the glycolysis pathway and causes oxidative damage by impairing protein synthesis [12,15,16].
To prevent Cd-induced oxidative stress, plants have stress tolerance mechanisms such as detoxification of ROS and MG, maintenance of nutrient homeostasis, and reduction of Cd uptake [6,12,16,17]. In addition, plants produce proline (Pro) or other compatible solutes to maintain proper water balance and stabilize the protein complexes for ionic and osmotic homeostasis [18,19].
Plants have an antioxidant defense system comprising non-enzymatic antioxidants, such as ascorbate (AsA), glutathione (GSH), phenolic compounds, alkaloids, non-protein amino acids, and α-tocopherols, and enzymatic antioxidants, such as superoxide dismutase (SOD), catalase (CAT), ascorbate peroxidase (APX), glutathione reductase (GR), monodehydroascorbate reductase (MDHAR), dehydroascorbate reductase (DHAR), glutathione peroxidase (GPX), and glutathione S-transferase (GST) to scavenge the overproduced ROS [2,20]. In addition, the glyoxalase enzymes [glyoxalase I (Gly I) and glyoxalase II (Gly II)] act coordinately with GSH to detoxify MG [15,21].
Regulating the antioxidant defense and glyoxalase systems, improving nutrient homeostasis, and reducing Cd uptake by applying an exogenous phytoprotectants might be an important strategy to reduce Cd-induced damage in rice seedlings. Manganese (Mn) is an essential trace element for plants and plays a role in several metabolic processes including photosynthesis, and respiration; synthesis of ATP, fatty acids, amino acids, lipids, proteins, and flavonoids; and activation of hormones [22,23]. Manganese also plays a vital role as cofactor in Mn-SOD and Mn-CAT, which participate in the plant's defense against oxidative stress [24]. Although the mechanism is not clear, it is also assumed that Mn act as a scavenger of O2·− and H2O2 [24]. Manganese is taken up by plants via an active transport mechanism as a divalent cation that can compete with divalent Cd, because they have some common routes of uptake and transportation [25,26]. This is the most important mechanism of Mn-induced reduction of Cd toxicity. Several studies revealed that supplemental Mn plays an important role in the adaptive responses of plant cells under environmental stresses [27–32]. Considering the strategies discussed, our present study was conducted to investigate the role of supplemental Mn in improving the antioxidant defense and glyoxalase systems, nutrient homeostasis, and Cd uptake reduction capacity in Cd-stressed rice seedlings.
2 Materials and methods
2.1 Plant materials and treatments
Rice (Oryza sativa L. cv. BRRI dhan29) seeds were surface-sterilized with 70% ethanol for 8–10 min, followed by washing several times with sterilized distilled water and soaking in distilled water in a dark place for 48 h. The imbibed seeds were then sown on plastic nets floating on distilled water in 250-mL plastic beakers kept in the dark at 28 ± 2 °C for 72 h. The uniformly germinated seeds were then transferred to a growth chamber (light, 350 μmol photon m−2·s−1; temperature, 25 ± 2 °C; relative humidity, 65–70%) with the same pot providing a diluted (5000 times) commercial hydroponics nutrient solution (Hyponex, Japan). The nutrient solution contained 8% N, 6.43% P, 20.94% K, 11.8% Ca, 3.08% Mg, 0.07% B, 0.24% Fe, 0.03% Mn, 0.0014% Mo, 0.008% Zn, and 0.003% Cu. The nutrient solutions were renewed twice a week. Each pot contained approximately 60 seedlings. Fourteen-day-old seedlings were exposed to Cd-stress (0.3 mM CdCl2) in the presence and absence of supplemental Mn (0.3 mM MnSO4) with nutrient solution to verify the role of exogenous Mn under a Cd-stress condition. Control plants were grown in Hyponex solution only. Our experiment consisted of four treatments as follows: control, 0.3 mM MnSO4 (Mn), 0.3 mM CdCl2 (Cd), and 0.3 mM CdCl2 + 0.3 mM MnSO4 (Cd + Mn). The experiment was repeated three times under the same conditions. Data were taken after three days of treatment.
2.2 Observation of cadmium toxicity symptoms and seedlings growth
Seedling growth and Cd toxicity symptoms in rice seedlings were determined by careful observation and by measuring fresh weight (FW) and dry weight (DW). For DW, seedlings were oven-dried at 70 °C for 48 h. The plant height was measured from the base of the shoot to the tip of the longest leaf.
2.3 Determination of the relative water content in leaves
The relative water content (RWC) in the leaves was measured according to the method of Barrs and Weatherley [33]. Leaf laminas were weighed [fresh weight (FW)] then placed immediately between two layers of filter paper and immersed in distilled water in a Petri dish for 24 h in a dark place. The turgid weight (TW) was measured after gently removing the excess water with a paper towel. The dry weight (DW) was measured after 48 h of oven-drying at 70 °C. Finally, RWC was determined using the following formula:
2.4 Determination of the Cd and mineral nutrient contents
The contents of Cd, Mn and of other mineral nutrients such as potassium (K), calcium (Ca) and magnesium (Mg) were determined by using an atomic absorption spectrophotometer (Z-5000; Hitachi, Japan). The plant samples were oven-dried at 70 °C for 48 h. The dried samples from the roots and shoots (0.1 g) were ground and digested separately with an acid mixture at 70 °C for 48 h. The acid mixture consisted of HNO3:HClO4 (5:1 v/v).
2.5 Determination of the chlorophyll content
The chlorophyll (chl) content was measured according to Arnon [34] by homogenizing the leaf samples (0.5 g) with 10 mL of acetone (80% v/v) followed by centrifuging at 10,000 g for 10 min. Absorbance was measured with a UV–vis spectrophotometer at 663 and 645 nm for the chl a and chl b contents, respectively. The carotenoid content was also measured spectrophotometrically at a wavelength of 470 nm.
2.6 Determination of the phenolic compounds
The total phenolic content was measured following the method of Ashraf et al. [35] with a modification. The leaf samples (0.5 g) were homogenized with 80% (v/v) acetone followed by centrifugation at 10,000 g for 10 min. The supernatant (50 μL) was mixed with Folin phenol reagent, 7% Na2CO3 and distilled water, the total volume being 5 mL, and the absorbance was measured at 750 nm. The total phenolic content was measured by comparing with a gallic acid standard.
2.7 Determination of the proline content
The proline content was determined according to Bates et al. [36]. Leaf samples (0.5 g) were homogenized in 5 mL 3% sulfo-salicylic acid and the homogenate was centrifuged at 11,500 g for 12 min. The supernatant (1 mL) was mixed with 1 mL of glacial acetic acid and 1 mL acid ninhydrin. After 1 h of incubation at 100 °C, the mixture was cooled. The developed color was extracted with 2 mL of toluene and the optical density of the chromophore was observed spectrophotometrically at 520 nm. The proline content was determined by comparing with a standard curve of known concentration of Pro.
2.8 Histochemical detection of ROS (O2·−, H2O2) generation in the leaves; lipid peroxidation and membrane damage in the roots
Localization of O2·− in leaves was detected following Chen et al. [37], with a slight modification. Leaves were immersed in a 0.1% solution of nitroblue tetrazolium chloride (NBT) and incubated at 25 °C. After 12 h, the incubated leaves were immersed in boiling ethanol (90%) for 15 min to decolorize the leaves and reveal the dark blue spots produced by the reaction of NBT and O2·−.
The localization of H2O2 was detected according to the method of Thordal-Christensen et al. [38], with a minor modification. The leaves were incubated at 25 °C in a solution containing 1 mg·mL−1 of 3,3-diaminobenzidine (DAB) prepared in HCl-acidified (pH 3.8) water. After 12 h of incubation, the leaves were boiled in ethanol (90%) for 15 min to reveal the reddish brown spots produced by the reaction of H2O2 and DAB.
The lipid peroxidation in the roots was determined by histochemical staining using Schiff's reagent with the modification of Srivastava et al. [17]. Lipid peroxidation-originated aldehydes were detected after 30 min of staining when the roots changed color to pink-red. The stained roots were rinsed with a sulfite solution (0.5% [w/v] K2S2O5 in 0.05 M HCl) and dipped in the same solution for 10 min to retain the stained color. The loss of plasma membrane integrity in the roots was measured with the slight modification of Schützendübel et al. [39] by histochemical staining using a 0.25% aqueous Evan's blue solution. After 40 min, the roots were rinsed with distilled water, and membrane damage was revealed using glycerine.
2.9 Determination of lipid peroxidation and hydrogen peroxide levels
The level of lipid peroxidation was measured by estimating malondialdehyde (MDA) following the method of Heath and Packer [40]. The malondialdehyde content was measured by observing the difference in absorbance at 532 nm using an extinction coefficient of 155 mM−1·cm−1 and expressed as nmol of MDA g−1 FW. The hydrogen peroxide content was determined according to Yu et al. [41] by observing the absorbance at 410 nm using an extinction coefficient of 0.28 μM−1·cm−1.
2.10 Determination of the methylglyoxal content
Methylglyoxal was measured following the method of Wild et al. [42] by extracting plant samples in 5% perchloric acid. After centrifuging the homogenized leaf tissues at 11,000 g for 10 min, the supernatant was decolorized by adding charcoal. The decolorized supernatant was neutralized by adding saturated Na2CO3 and used to estimate MG by adding sodium dihydrogen phosphate and N-acetyl-l-cysteine to a final volume of 1 mL. The absorbance was recorded after 10 min at 288 nm and the MG content was calculated using a standard curve of known concentration of MG.
2.11 Determination of ascorbate and glutathione
Rice leaves (0.5 g) were homogenized in 3 mL of ice-cold 5% meta-phosphoric acid containing 1 mM EDTA using a mortar and a pestle. The homogenates were centrifuged at 11,500x g for 15 min at 4 °C, and the collected supernatants were used according to the method of Dutilleul et al. [43] with minor modifications to determine total and reduced AsA. After neutralizing the supernatant with 0.5 M potassium phosphate (K-P) buffer (pH 7.0), the oxidized fraction was reduced with 0.1 M dithiothretitol. The total and reduced AsA contents were assayed spectrophotometrically at 265 nm in a 100 mM K-P buffer (pH 7.0) with 1.0 U of ascorbate oxidase (AO). To calculate ascorbate, a specific standard curve of AsA was used. Dehydroascorbate (DHA) was measured using the formula DHA = total AsA − reduced AsA. Reduced glutathione (GSH), oxidized glutathione or glutathione disulfide (GSSG), and total glutathione (GSH + GSSG) was determined according to the method of Griffiths [44] based on enzymatic recycling. Glutathione was measured using the formula GSH = total GSH − GSSG. Glutathione was removed by 2-vinylpyridine derivatization to determine GSSG.
2.12 Determination of proteins
The protein concentration was measured according to Bradford [45] using bovine serum albumin (BSA) as a protein standard.
2.13 Enzyme extraction and assays
Rice leaves (0.5 g) were homogenized in 50 mM ice-cold K-P buffer (pH 7.0) containing 100 mM of KCl, 1 mM ofascorbate, 5 mM of β-mercaptoethanol, and 10% (w/v) of glycerol using a pre-cooled mortar and pestle. The homogenates were centrifuged two times at 11,500 g for 15 min, and the supernatants were used to determine the protein content and the enzyme activity. All procedures were performed between 0 and 4 °C.
Lipoxygenase (LOX, EC: 1.13.11.12) activity was determined according to the method of Doderer et al. [46] using linoleic acid as a substrate solution. The increased absorbance was observed at 234 nm and the activity was calculated using an extinction coefficient of 25 mM−1·cm−1.
Ascorbate peroxidase (APX, EC: 1.11.1.11) activity was determined according to Nakano and Asada [47] by observing the decreased absorbance at 290 nm for 1 min and using an extinction coefficient of 2.8 mM−1·cm−1.
Monodehydroascorbate reductase (MDHAR, EC: 1.6.5.4) activity was assayed using the method of Hossain et al. [48] using an extinction coefficient of 6.2 mM−1·cm−1.
Dehydroascorbate reductase (DHAR, EC: 1.8.5.1) activity was determined according to the method of Nakano and Asada [47] by observing the change in absorbance at 265 nm for 1 min using an extinction coefficient of 14 mM−1·cm−1.
Glutathione reductase (GR, EC: 1.6.4.2) activity was determined according to the method of Foyer and Halliwell [49] by monitoring the decreased absorbance at 340 nm using an extinction coefficient of 6.2 mM−1·cm−1.
Glutathione S-transferase (GST, EC: 2.5.1.18) activity was measured as described by Hossain et al. [50]. The activity was calculated by observing the increased absorbance at 340 nm for 1 min and using an extinction coefficient of 9.6 mM−1·cm−1. The reaction mixture contained 100 mM Tris-HCl buffer (pH 6.5), 1.5 mM GSH, and 1 mM 1-chloro-2,4-dinitrobenzene (CDNB), and enzyme solution.
Glutathione peroxidase (GPX, EC: 1.11.1.9) activity was determined according to Elia et al. [51] by monitoring the change in absorbance at 340 nm for 1 min using an extinction coefficient of 6.62 mM−1·cm−1.
Superoxide dismutase (SOD, EC: 1.15.1.1) activity was measured based on the xanthine–xanthine oxidase system following the method of El-Shabrawi et al. [52].
Catalase (CAT, EC: 1.11.1.6) activity was measured as described by Hasanuzzaman and Fujita [53] using an extinction coefficient of 39.4 mM−1·cm−1.
Glyoxalase I (Gly I, EC: 4.4.1.5) and glyoxalase II (Gly II, EC: 3.1.2.6) activities were determined as described by Hasanuzzaman and Fujita [53] using an extinction coefficient of 3.37 and 13.6 mM−1·cm−1, respectively.
2.14 Statistical analysis
The data were subjected to analysis of variance (ANOVA) and the mean differences were compared by Fisher's LSD using XLSTAT v.2015 software [54]. Differences at P ≤ 0.05 were considered significant.
3 Results
3.1 Phenotypic appearance and plant growth
Differences in phenotypic appearance of the seedlings were easily comparable by Cd exposure (Fig. 1). Cadmium treatment caused rolling, necrosis, burning, and yellowing of leaves. Supplemental Mn improved the phenotype of the Cd-stressed seedlings (Fig. 1). Exposure of the rice seedlings to Cd also decreased plant growth in terms of plant height, FW, and DW, which were partially reversed by supplemental Mn (Table 1). However, exogenous application of Mn to the non-stressed seedlings did not change phenotypical appearance.

Phenotypic appearance of rice seedlings under different treatments. Here, Mn and Cd indicate 0.3 mM MnSO4 and 0.3 mM CdCl2, respectively.
Effect of exogenous Mn on growth, leaf RWC and proline content in the leaves of Cd-stressed seedlings.
Treatment | Plant height (cm) | Fresh weight (mg·seedling−1) |
Dry weight (mg·seedling−1) |
Leaf RWC (%) | Proline content (μmol·g−1 FW) |
Control | 19.97 ± 0.24 a | 87.13 ± 3.95 a | 16.69 ± 1.65 a | 97.59 ± 1.32 a | 0.24 ± 0.02 c |
Mn | 19.79 ± 0.19 a | 86.42 ± 3.24 a | 16.27 ± 1.26 a | 97.23 ± 1.03 a | 0.24 ± 0.03 c |
Cd | 15.98 ± 0.15 c | 62.24 ± 3.41 c | 12.63 ± 0.86 b | 81.22 ± 3.46 c | 0.54 ± 0.04 a |
Cd + Mn | 17.46 ± 0.12 b | 74.10 ± 4.95 b | 15.31 ± 0.86 a | 92.43 ± 2.14 b | 0.30 ± 0.03 b |
3.2 Effect on the leaf relative water content and proline content
The relative water content in leaves decreased with Cd-induced stress, but was considerably recovered by exogenous Mn (Table 1). A marked increased in the Pro content was observed in the Cd-treated seedlings, compared with controls (Table 1). Exogenous application of Mn decreased the Pro content in the Cd-exposed seedlings, compared with the seedlings treated with Cd alone. However, supplemental Mn in control seedlings had no effect on the RWC and Pro contents of non-stressed seedlings.
3.3 Effect on chlorophyll and carotenoid contents
Compared with the control seedlings, the chl a, chl b, chl (a + b) and carotenoid contents decreased in the Cd-stressed seedlings, but were restored by supplemental Mn (Table 2).
Effect of exogenous Mn on chlorophyll, carotenoid and total phenol contents of Cd-stressed seedlings.
Treatment | chl a (mg·g−1 FW) |
chl b (mg·g−1 FW) |
chl (a + b) (mg·g−1 FW) |
Carotenoid (mg·g−1 FW) |
Total phenol content (μmol·g−1 FW) |
Control | 1.18 ± 0.022 | 0.48 ± 0.014 a | 1.65 ± 0.015 a | 0.36 ± 0.011 a | 22.12 ± 2.4 b |
Mn | 1.19 ± 0.014 | 0.48 ± 0.020 a | 1.67 ± 0.029 a | 0.36 ± 0.009 a | 23.97 ± 1.89 b |
Cd | 0.86 ± 0.019 | 0.36 ± 0.016 c | 1.22 ± 0.034 c | 0.27 ± 0.011 c | 32.92 ± 2.06 a |
Cd + Mn | 1.07 ± 0.007 | 0.42 ± 0.013 b | 1.50 ± 0.030 b | 0.33 ± 0.009 b | 25.64 ± 2.55 b |
3.4 Effect on total phenolic compounds
Treating the seedlings with Cd considerably increased the total phenolic content, compared with the control seedlings, whereas Mn supplementation reduced the total phenolic content compared with the seedlings treated with Cd alone (Table 2). However, applying Mn without Cd-stress did not affect the total phenolic content of the seedlings.
3.5 Effect on Cd uptake and nutrient homeostasis
The Cd-induced stress resulted in higher accumulation of Cd, which was reversed by exogenous Mn (Fig. 2). Exogenous application of Mn to the Cd-stressed seedlings decreased Cd uptake by 40 and 60% in the roots and shoots, respectively, compared with seedlings treated with Cd alone. The decrease in Cd accumulation by supplemental Mn was higher in the shoots compared with the roots, which indicated that Cd translocation from the roots to the shoots also decreased.

Effect of exogenous Mn on Cd and Mn content in the roots (A, C) and shoots (B, D) of Cd-stressed seedlings. Here, Mn and Cd indicate 0.3 mM MnSO4 and 0.3 mM CdCl2, respectively. Means (± SD) were calculated from three replicates for each treatment. Bars with different letters are significantly different at P ≤ 0.05 applying Fisher's LSD test.
Treatment of the seedlings with Cd decreased Mn uptake in both the roots and shoots (Fig. 2). However, supplemental Mn increased Mn uptake compared with control and Cd-treated seedlings. The mineral nutrient content decreased in the roots (K and Mg) and the shoots (Ca and Mg) of the Cd-stressed seedlings, compared with control seedlings (Table 3). Simultaneous application of Mn in Cd-treated seedlings improved the K and Mg content, compared with seedlings treated with Cd alone.
Effect of exogenous Mn on mineral nutrient content (K, Mg and Ca) of Cd-stressed seedlings.
Treatment | K content (μmol·g−1 DW) | Mg content (μmol·g−1 DW) | Ca content (μmol·g−1 DW) | |||
Root | Shoot | Root | Shoot | Root | Shoot | |
Control | 272.57 ± 2.81 a | 343.58 ± 4.02 a | 72.74 ± 2.51 a | 116.91 ± 2.68 a | 50.56 ± 1.38 a | 181.12 ± 2.95 a |
Mn | 270.87 ± 3.11 a | 341.55 ± 4.36 a, b | 71.31 ± 3.71 a | 115.62 ± 2.89 a | 47.38 ± 1.63 a, b | 165.55 ± 3.78 b |
Cd | 162.93 ± 2.95 c | 333.83 ± 6.85 b | 36.77 ± 2.40 c | 108.73 ± 3.31 b | 48.06 ± 2.24 a, b | 148.84 ± 2.93 c |
Cd + Mn | 239.93 ± 4.89 b | 335.23 ± 4.65 a, b | 50.12 ± 3.04 b | 107.86 ± 3.46 b | 44.27 ± 5.10 b | 141.64 ± 2.68 d |
3.6 Effect on ROS generation and lipid peroxidation
Overproduction of ROS was observed in the Cd-affected seedlings, but was reversed by simultaneous application of Mn. Cadmium exposure resulted in 69% higher H2O2 production, compared with control seedlings (Fig. 4B). Histochemical staining showed overproduction of O2·− and H2O2 revealed by dark blue and brown spots, respectively, in Cd-affected seedlings (Fig. 3A and B). However, supplementation of Mn to Cd-treated seedlings played a positive role in reducing ROS.
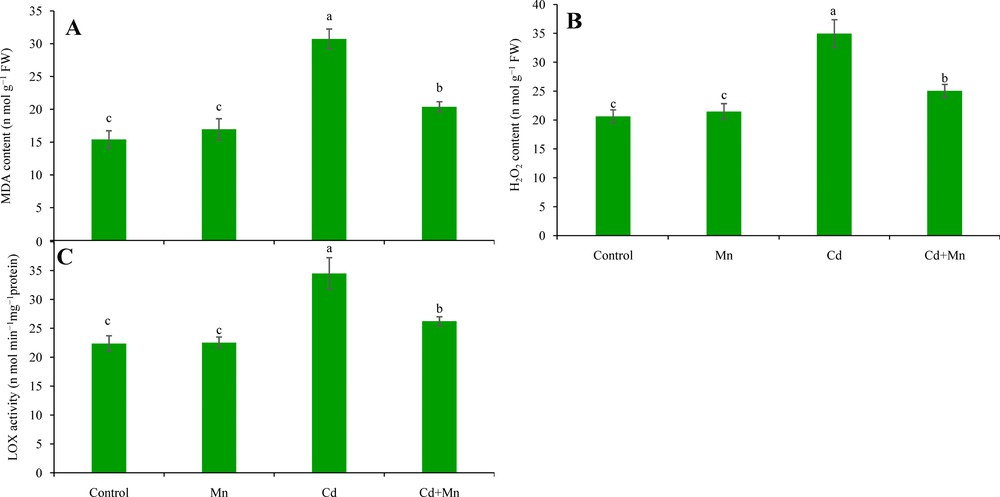
Effect of exogenous Mn on lipid peroxidation (MDA content) (A), H2O2 content (B) and LOX activity (C) in the leaves of of Cd-stressed seedlings. Here, Mn and Cd indicate 0.3 mM MnSO4 and 0.3 mM CdCl2, respectively. Means (± SD) were calculated from three replicates for each treatment. Bars with different letters are significantly different at P ≤ 0.05 applying Fisher's LSD test.
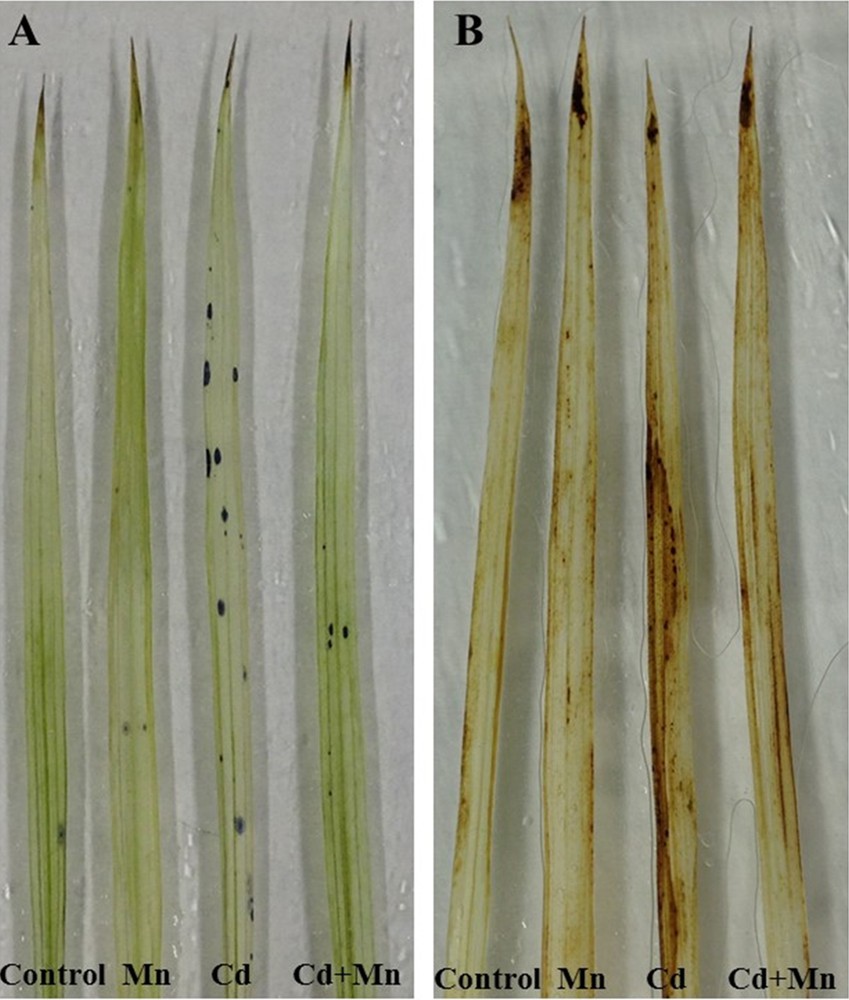
Histochemical detection of O2•− (A) and H2O2 (B) in the leaves of Cd-stressed seedlings. Here, Mn and Cd indicate 0.3 mM MnSO4 and 0.3 mM CdCl2, respectively.
The increased ROS content resulted in oxidative damage in the seedlings, leading to increased lipid peroxidation and membrane damage of cells, indicated by the MDA content and root staining. Compared with the control seedlings, a marked increase in the MDA content was observed in the leaves of Cd-stressed seedlings (Fig. 4A). Higher lipid peroxidation and loss of plasma membrane integrity were observed in the roots of Cd-affected seedlings, as revealed by the roots changing color to an intense pink-red and dark blue, respectively (Fig. 5A and B). Supplementation of Mn to Cd-treated seedlings reduced oxidative damage, which was revealed by a decrease in the MDA content and a reduction in the formation of an intense color.

Histochemical detection of lipid peroxidation (A) and loss of plasma membrane integrity (B) in the roots of Cd-stressed seedlings. Here, Mn and Cd indicate 0.3 mM MnSO4 and 0.3 mM CdCl2, respectively.
3.7 Effect on LOX activity
The seedlings treated with Cd had increased LOX activity, compared with control seedlings (Fig. 4C). In contrast, exogenous Mn decreased LOX activity in Cd-exposed seedlings.
3.8 Effect on AsA and GSH
The seedlings exposed to Cd showed lower AsA content and AsA/DHA ratio, but higher DHA content, compared with control seedlings (Fig. 6A–C). However, supplemental Mn partly increased the AsA content and AsA/DHA ratio and decreased the DHA content in Cd-affected seedlings.

Effect of exogenous Mn on AsA content (A), DHA content (B), AsA/DHA ratio (C) GSH content (D), GSSG content (E), and GSH/GSSG ratio (F) in the leaves of Cd-stressed seedlings. Here, Mn and Cd indicate 0.3 mM MnSO4 and 0.3 mM CdCl2, respectively. Means (± SD) were calculated from three replicates for each treatment. Bars with different letters are significantly different at P ≤ 0.05 applying Fisher's LSD test.
Compared with control seedlings, Cd-induced oxidative stress resulted in higher GSH and GSSG contents with a lower GSH/GSSG ratio (Fig. 6D–F). Application of Mn decreased the GSSG content and increased the GSH/GSSG ratio, while the GSH content remain unchanged.
3.9 Effect on antioxidant enzymes
Exposure to Cd increased the APX, MDHAR, and GR activities, but decreased the DHAR activity in Cd-treated seedlings, compared with control (Fig. 7A–D). In contrast, Mn supplementation to Cd-stressed seedlings increased the MDHAR and DHAR activities, while GR activity remained similar compared to seedlings treated with Cd alone.

Effect of exogenous Mn on APX (A), MDHAR (B), DHAR (C) and GR (D) activity in the leaves of Cd-stressed seedlings. Here, Mn and Cd indicate 0.3 mM MnSO4 and 0.3 mM CdCl2, respectively. Means (± SD) were calculated from three replicates for each treatment. Bars with different letters are significantly different at P ≤ 0.05 applying Fisher's LSD test.
Compared with the control seedlings, the activities of GPX and SOD increased, but GST and CAT activity decreased in the Cd-affected seedlings (Fig. 8A–D). However, Mn supplementation to Cd-exposed seedlings further stimulated SOD activity, while GPX and GST activities remained the same as those recorded with seedlings treated with Cd alone. Catalase activity also increased in Cd-stressed seedlings with Mn supplementation.

Effect of exogenous Mn on GPX (A), GST (B), SOD (C) and CAT (D) activity in the leaves of Cd-stressed seedlings. Here, Mn and Cd indicate 0.3 mM MnSO4 and 0.3 mM CdCl2, respectively. Means (± SD) were calculated from three replicates for each treatment. Bars with different letters are significantly different at P ≤ 0.05 applying Fisher's LSD test.
3.10 Effect on glyoxalase system
Application of Cd to rice seedlings increased the MG content with increased Gly I and decreased Gly II activities (Fig. 9A–C). Exogenous application of Mn decreased the MG content and increased the Gly I and Gly II activities, compared with Cd-treated seedlings.

Effect of exogenous Mn on Gly I (A) and Gly II activity (B), and MG content (C) in the leaves of Cd-stressed seedlings. Here, Mn and Cd indicate 0.3 mM MnSO4 and 0.3 mM CdCl2, respectively. Means (± SD) were calculated from three replicates for each treatment. Bars with different letters are significantly different at P ≤ 0.05 applying Fisher's LSD test.
4 Discussion
4.1 Nutrient homeostasis
Plants exposed to a Cd-contaminated growth medium cause higher Cd accumulation and lower nutrient uptake [6,9]. In addition, Cd enters the roots and is transported inside the plant using the same transporters as those used by many essential elements, including Ca, Mg, Mn and zinc (Zn). Consequently, Cd competes with these elements during uptake and further translocation from the roots to the upper parts of plants in Cd-contaminated growth medium [32,55]. In the present study, rice seedlings exposed to Cd resulted in higher Cd accumulation and lower Mn uptake in both the roots and shoots. The content of the other mineral nutrients also decreased in the roots (K and Mg) and the shoots (Ca and Mg) under a Cd-stressed condition. Higher Cd accumulation disrupted nutrient homeostasis by reducing mineral uptake, which is in agreement with Sandalio et al. [9] and Ahmad et al. [6]. However, simultaneous application of Mn to Cd-treated seedlings decreased Cd uptake and increased mineral nutrient uptake including Mn as well as K and Mg. The reduction of Cd uptake and translocation from the roots to shoots might be due to a competition with Mn, because Cd and Mn use some common transporters for their uptake and translocation in plants. This lower accumulation of Cd as well as its translocation in the upper parts of plant were also reported in previous studies [31,32], which showed that supplemental Mn reduced Cd accumulation and increased Mn uptake.
4.2 Physiological attributes
Higher accumulation of Cd disturbs the plant–water relation, which causes loss of water from cells, reduces RWC, and inhibits growth [6,17]. In the present study, treatment of rice seedlings with Cd resulted in higher Cd accumulation, lower RWC, and reduction of growth in terms of FW, DW, and plant height, which were reversed by Mn supplementation to Cd-treated seedlings. These results are supported by Sebastian and Prasad [32], who showed that supplemental Mn reversed Cd-induced growth inhibition by reducing Cd uptake. Water loss caused by toxic metals and other abiotic stresses reduces RWC and increases Pro accumulation [16,56]. Being an osmoprotectant, Pro plays a vital role in osmoregulation under abiotic stress [57,58]. The present study showed lower RWC and higher accumulation of Pro under a Cd-stress condition were reversed by Mn supplementation. The lower RWC and higher Pro accumulation indicated a water imbalance under Cd-stress conditions, which were partially recovered by Mn supplementation. These results are also supported by previous findings [16,59], which reported that Cd-stress disturbed water balance by decreasing RWC and consequently increased the Pro content. Toxic metals including Cd reduce the chl content and cause oxidative damage to photosynthetic pigments [6,60,61]. Exogenous application of Mn increased the content of chl and carotenoid in rice seedlings under Cd-stress. These results are consistent with previous findings [27,32] that reported that supplemental Mn increased the chl content under abiotic stress conditions.
4.3 ROS generation, lipid peroxidation, and oxidative stress
Although Cd does not directly play a role in ROS generation via a Haber–Weiss or Fenton reaction, it can cause oxidative damage by disrupting the electron transport chain [13] or the metabolism of essential elements [14]. Cadmium can cause oxidative stress indirectly by activating NADPH oxidase, which is present in plasma membranes, increasing the production of O2·− and H2O2 [62]. The oxidative stress resulting from higher ROS production increases LOX activity, which also causes lipid peroxidation [63]. Higher production of ROS under abiotic stress conditions causes lipid peroxidation, protein oxidation, and enzyme inhibition, and eventually leads to cell death [20]. In our study, exposure to Cd resulted in higher ROS production (O2·− and H2O2), lipid peroxidation (indicated by the MDA content), LOX activation and loss of plasma membrane integrity. However, the external Mn reduced the oxidative damage by reversing ROS production, LOX suppression, loss of plasma membrane integrity, lipid peroxidation and Cd uptake. These results are also supported by Sebastian and Prasad [32], who showed that supplemental Mn reduced Cd-induced oxidative stress by reducing ROS production and lipid peroxidation and Cd accumulation.
4.4 Antioxidant defense system
The non-enzymatic antioxidant components such as AsA, GSH and phenolic compounds play a potential role in protecting cell organelles and biomolecules from oxidative damage by direct scavenging of ROS [20]. The steady ratio of AsA and DHA, and the stable ratio of GSH and GSSG are crucial in maintaining a cellular redox state under abiotic stress conditions [2,64]. Our results showed that Cd-stress decreased the AsA content and the AsA/DHA ratio and increased the DHA content because of increased oxidation during the scavenging of overproduced ROS. In our experiment, we also found that simultaneous application of Mn to the Cd-treated seedlings improved the AsA content and AsA/DHA ratio and decreased the DHA content through facilitating AsA regeneration by increased MDHAR and DHAR activities. These results are consistent with previous studies [16,17], which showed that Cd-induced stress decreased the AsA content and the AsA/DHA ratio. These results are also supported by Sebastian and Prasad [32], who reported that supplemental Mn improved the AsA content under Cd-stress conditions. In the present study, the levels of GSH and GSSG increased with increased GR activity and decreased GST activity under Cd-stress conditions, which remained unchanged with Mn supplementation. The GSSG content increased because of increased oxidation of GSH during ROS detoxification, which declined with Mn supplementation because exogenous Mn reduced ROS production. These results are in agreement with previous studies [12,65], which noted that the GSH content increased under certain levels of toxic metal stress, including Cd. The other non-enzymatic component, phenolic compounds, are secondary metabolites that can increase or decrease under abiotic stress and exhibit antioxidant properties by scavenging ROS [66]. In the present study, the total phenolic content increased under Cd-stress because of higher ROS production, which decreased with Mn supplementation as exogenous Mn reduced Cd uptake and ROS production.
In the AsA-GSH cycle, the antioxidant enzymes (APX, MDHAR, DHAR, and GR) work together with AsA and GSH to detoxify ROS and further recycle AsA and GSH [2,67,68]. Ascorbate peroxidase converts H2O2 to H2O via the oxidation of AsA to MDHA. Regeneration of AsA is maintained by the activity of MDHAR and DHAR using NADPH and GSH, which is necessary to maintain the antioxidative potential of AsA. On the other hand, GR together with GPX and DHAR also regenerate the antioxidant component GSH to maintain the redox cellular balance [17]. In our experiment, Cd-induced oxidative stress decreased the AsA content and DHAR activity, and increased the APX, MDHAR, and GR activities and the GSH content. These findings are corroborated by previous findings [17,59,69], which showed that Cd-stress stimulated the APX, MDHAR, and GR activities, and degraded DHAR activity. Degradation and stimulation of these components under stress indicated that they correlate with oxidative stress [68,70]. Upregulation of APX activity might correlate with higher H2O2 and lower AsA contents because APX detoxifies H2O2 using AsA. However, simultaneous Mn supplementation stimulated the MDHAR and DHAR activities and increased the AsA contents while the APX and GR activities remained unchanged. The increased activities of MDHAR and DHAR correlate with increased AsA content and decreased DHA content because AsA is regenerated from DHA by the activities of MDHAR and DHAR. These findings are supported by previous findings [12,16,17] that showed that MDHAR and DHAR activities increased with increased AsA content and decreased DHA content, which helped in ROS detoxification under Cd-stress conditions.
Under abiotic stress conditions, SOD is considered the first line of enzymatic defense in controlling ROS, SOD converts O2·− to H2O2 [71], and H2O2 is readily converted to H2O and O2 by CAT [72]. In our study, Cd-treated seedlings showed increased SOD activity and decreased CAT activity, which might result from conversion O2·− to H2O2. These results are in agreement with previous results [6,73] in which the activities of SOD and CAT increased under Cd-stress. In the present study, supplementation with Mn to the Cd-treated seedlings further stimulated the CAT and SOD activities. The Mn-induced higher SOD and CAT activities might be because of the involvement of Mn as a cofactor in Mn-SOD and Mn-CAT, respectively, which may also be responsible for decreased ROS contents in Cd-treated rice seedlings. These results are consistent with the findings of Sebastian and Prasad [32], who showed supplemental Mn upregulated SOD activity under Cd-stress conditions.
Glutathione peroxidase and GST play a pivotal role in protecting plants from oxidative stress by forming a GSH-dependent conjugation of lipid hydroperoxides and endobiotic substrates [74]. Glutathione peroxidase uses GSH as a substrate to scavenge H2O2 and lipid hydroperoxides [75]. Together with GSH, GST produces less toxic and water-soluble conjugates by catalyzing the binding of different xenobiotics and their electrophilic metabolites [76]. In the present study, exposure to Cd resulted in higher GPX and lower GST activities, which correlated with the higher H2O2 content. Our findings corroborate previous reports by Srivastava et al. [17] and Rahman et al. [59]. However, Mn supplementation did not change GPX and GST activities.
4.5 Glyoxalase system
Plants exposed to environmental stresses generate high amounts of cytotoxic MG [15,16,58]. However, it is detoxified by the sequential action of the thiol-dependent enzymes Gly I and Gly II. Stimulation of Gly I and Gly II increases tolerance to abiotic stresses by increasing MG detoxification in many plant species [21,77,78]. In the current study, Cd-treated rice seedlings showed higher MG production along with increased Gly I and decreased Gly II activities, which is consistent with previous findings [16,59]. Exogenous application of Mn decreased the MG content and increased the Gly I and Gly II activities compared with the Cd-treated rice seedlings. The decreased MG content with Mn supplementation was associated with increased Gly I and Gly II activities. These results are supported by previous studies in which the stimulation of the Gly I and Gly II activities detoxified MG after application of exogenous phytoprotectants under Cd-stress [16,59,79].
Our results suggested that Cd-induced stress caused oxidative stress by disrupting nutrient homeostasis, and the antioxidant defense and glyoxalase systems through higher Cd accumulation, ROS production, and MG formation, respectively. However, supplemental Mn in Cd-treated seedlings improved nutrient homeostasis by decreasing Cd uptake and its transportation to the upper parts of the plant. Supplemented Mn also improved ROS and MG detoxification by improving the antioxidant defense and glyoxalase systems in Cd-treated seedlings, which partially recovered Cd-induced damage. Lower accumulation of Cd in plants by supplemental Mn might be useful in reducing toxic metal accumulation in the edible parts of plant.
Contributions of the authors
AR conceived, designed, and performed the experiment and prepared the manuscript. KN actively participated in executing the experiment. MH designed the experiment and analyzed the data. MF conceived, designed and monitored the experiment.
Disclosure of interest
The authors declare that they have no competing interest.
Acknowledgements
This research was funded by the Ministry of Education, Culture, Sports, Science and Technology (MEXT), Japan. We thank Mr. Jubayer-Al Mahmud and Mr. Shahadat Hossain, Laboratory of Plant Stress Responses, Faculty of Agriculture, Kagawa University, Japan for the critical reading of the manuscript.