Abbreviations
SE
sieve elements
CCcompanion cells
ROSreactive oxygen species
EPWelectric propagation waves
PMplasma membrane
ERendoplasmic reticulum
PPUpore/plasmodesmata units
RFOmember of the raffinose oligosaccharide family
1 Introduction
Phloem and xylem are the two conducting tissues found in land plants and are closely associated in all organs. The phloem orchestrates long distance transport and allocation of a range of nutrients and signals that play an integrative role in nutrition, development and adaptation to stresses. This is achieved by mass flow through the sieve elements (SE) and by cell-to-cell transport from surrounding cells, companion cells (CC) and phloem parenchyma cells (PPC), which collectively coordinate collection, storage, uploading and downloading of information molecules and metabolites [1,2]. Exchanges with xylem are important and not restricted to water but will not be considered in this review. A major breakthrough was the discovery of a key role of phloem in long distance trafficking of macromolecules [3]. But strong evidence has accumulated that the phloem integrates a range of signalling pathways that recruits metabolites such as sugars or amino acids, phytohormones, proteins, RNAs, to maintain the capacity of the plant to make physiological adjustments or developmental responses based on nutrient availability and environmental constraints. This review focuses on the most recent studies that have explored the complexity of the networks regulating sugar transport and signalling and highlights the emerging questions on networks operating through the phloem. For more detailed descriptions of phloem biology, readers are directed to recent reviews on structures [2], sampling methods [4], phloem transcriptomes [5] and macromolecular trafficking [3,4,6].
2 A transport pathway for nutrients and long distance signals
Phloem functions differ according to organ location. At least three parts can be defined: collection phloem in source organs (minor veins), transport phloem (along the path from sources to sinks) and release phloem in sink organs [2]. Along this pathway the composition of the phloem sap may change because of release and retrieval of molecules [7], resulting in some cases from selective withdrawal or polymer trapping to reduce solute leakage [8]. Large surveys of metabolites and macromolecules that are mobile in sieve tubes were undertaken using different methods to collect phloem sap, using either EDTA-facilitated exudation, stylectomy (using severed stylets from phloem feeding insects) or bleeding in species that spontaneously exudate [4]. Special attention should be given to possible contaminations or artefacts due to the mode of collection of phloem sap exudates, resulting from leakage from other cell types, from degradation during the collect time or to wound response due to collect method [4,5]; however, these studies provided tremendous information on phloem sap content.
Phloem sap contains large amounts of carbohydrates, mainly in the form of sucrose. High carbohydrate concentrations in the sap fulfil two roles: a source of carbon skeletons either converted to glucose (energy) for growth or to polysaccharides for storage, and a driving force for sap movement according to the mass-flow model of Münch [9]. Sink organs rely heavily on the delivery of carbohydrates through the phloem for growth and development and for storage of polysaccharides. Besides sucrose, other sugars are found and sometimes may be as abundant as sucrose, depending on species. They include polyols and oligosaccharides of the raffinose family. Other nutrients, such as amino acids and organic acids, are also found [10,11]. Sucrose and glucose are also acting as signalling molecules [12]. Long distance transport of additional signal molecules, such as hormones, proteins and RNAs, is also largely documented [13]. Thus, the basic function of phloem as a conduit to deliver photoassimilates has evolved to a more general role as a path involved in long distance regulation of development and response to stress [13,14].
3 Apoplasmic loaders: key role of sucrose transporters
There are three recognized strategies of sucrose loading: apoplasmic and symplasmic, with or without polymer trapping [15]. In many herbaceous species, phloem loading of sugars occurs via an apoplastic pathway (Fig. 1) and this requires the activity of specific sugar transporters [16,17]. Typical apoplasmic loaders are Solanaceaous species. Arabidopsis is also a considered as an apoplasmic loader [18]. Most of our knowledge is based on studies carried out on uptake sucrose transporters. They cotransport sucrose with proton and use the energy stored in the transmembrane proton gradient established by the proton pumping plasma membrane ATPase (PM-H+/ATPase). In minor veins of apoplasmic species, the coupled activity of the PM-H+/ATPase and sucrose transporters raises the sucrose concentration in the phloem much above those found in the surrounding cells: the ratio goes from 4.5 in celery to 40 in common plantain [19].
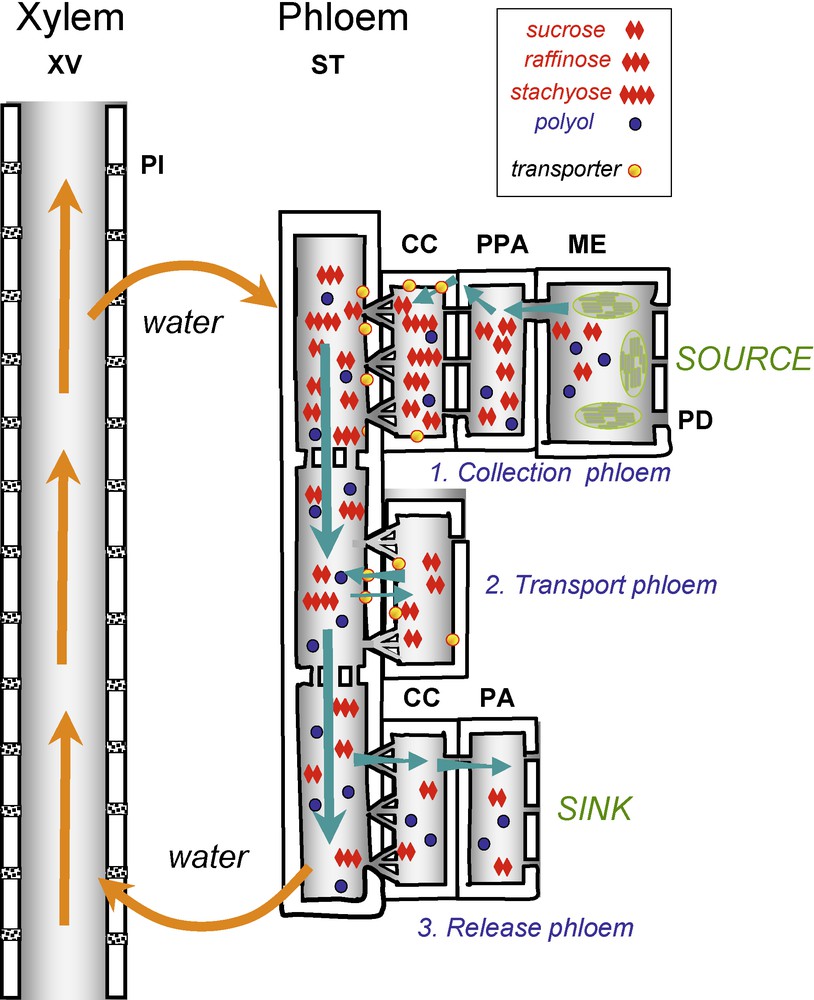
Schematic representation of sugar transport from source to sink organs. In mesophyll cells (ME) of apoplasmic loaders, sucrose or other sugars, such as polyols, produced from triose phosphates resulting from the Calvin cycle in chloroplasts, are transported from cell to cell through plasmodesmata to the phloem parenchyma cells (PPA) and then, by an unknown mechanism, are translocated into the apoplasm (collection phloem). Sucrose, as well as sugar alcohols, are taken up in the companion cell (CC)/sieve element (SE) complex by a sugar symporter (yellow circles) energized by proton pump ATPase in apoplasmic species. In symplasmic loaders, sugars are transported by passive diffusion from mesophyll cells to CC/SE complex via plasmodesmata (PD). Along the pathway between sources and sinks (transport phloem), the release of sugars is mostly apoplasmic. In terminal sinks (release phloem), the release of sugar is mostly symplasmic. In the phloem sieve elements, the movement of the sap is generated by a pressure-driven mass flow. Transport in the phoem requires an hydraulic coupling to the xylem water flow. Changes in xylem water potential are thus transmitted to the phloem, and in the root water is recircularized from phloem to xylem. This scheme can also apply to other substrates such as polyols and amino acids. XV: xylem vessels. ST: sieve tubes. CC: companion cells. PPC: phloem parenchyma cells. ME: mesophyll cells. PI: half-bordered pit. PD: plasmodesmata. Branched plasmodesmatas occur between CC and SE.
4 Loading sites in apoplasmic loaders: a confused picture
In the minor veins in source organs, the site of loading, as defined by the cells in which sucrose transporters acting on uptake are located, is still a matter of debate and no definite pattern has been found so far. In Solanaceaous species, sucrose transporters of the SUT1/SUC2 type were localized to the plasma membrane of sieve elements [20–23] whereas in Arabidopsis and Plantago major [24,25], they were located to companion cells. In each case, the mRNAs were located in companion cells by in situ hybridization. Also, the turnover of the sucrose transporters was reported to be rapid [20,26].
However, in Solanaceous species, antibodies raised against a sequence common to the SUT1 transporters of tobacco, potato and tomato, localized them to companion cells, in addition to xylem parenchyma cells [27], suggesting a different site of accumulation than those initially reported (i.e. sieve elements). An earlier study reported that a translation fusion of the tomato SUT1 gene with the GFP reporter gene, when expressed in transgenic plants, was detected in companion cells but not in sieve elements [28]. At that time, the authors concluded that, in contrast to the native transporter, the fusion protein was not able to cross the plasmodesmata between companion cells and sieve element. Finally, a localization outside the phloem tissue was even reported for some of them. For example, using transgenic plants expressing a fusion between the SUT1 gene (including promoter, introns and exons) and the GUS reporter gene, the site of transcription of the tomato SUT1 transporter gene was reported in trichomes and guard cells, in addition to high expression in both abaxial and adaxial phloem [29]. In this case, the authors showed a major role of introns in this localization suggesting some transcriptional regulations [29]. Again, the results differed from those obtained by immunolocalization [27].
To conciliate such observations, it would be interesting to determine whether post-translation modifications may affect transporter stability and localization. Indeed, oligomerisation and phosphorylation can be prerequisites for plasma membrane targeting of some plant membrane proteins, as reported for aquaporins [30]. Whether such changes may affect trafficking from companion cells to sieve elements is unknown. However, the discrepancies reported in the literature suggest that yet unidentified factors affect localization. Depending on species, other sugar uptake patterns were reported. For example, in Alansoa [31] and in celery [32], sucrose and mannitol transporters, respectively, were located both in phloem parenchyma cells and in companion cells, suggesting a role of phloem parenchyma in addition to those of CC in sugar uptake.
Interestingly, the transporters involved in sucrose uptake in source leaves, such as AtSUC2 in Arabidopsis, StSUT1 in potato or ZmSUT1 in maize, were also located in transport and release phloem [33–35], suggesting an additional role either in retrieval from the apoplasm or in release of sucrose.
5 Functional validation of sucrose uptake sites by reverse genetics
To address the respective role of the different cell types in uptake, the use of reverse genetic approaches might be useful. Mutant plants, in which the expression of the genes coding for SUT1/SUC2 transporters is affected, are available [34,36–38]. In Arabidopsis, the role of AtSUC2 in phloem loading of sucrose and the development of sink organs was investigated by the study of several Arabidopsis suc2 knock-out lines that did not grow at the homozygous state [39,40]. A weak allele of suc2, named pho3 because of low phosphate accumulation in the mutant, was also described [41,42]. Such mutants accumulated large amounts of sugars and starch, as well as anthocyanins [39,40,42]. A survey of gene expression in the pho3 mutant showed that the expression of genes involved in photosynthesis did not change, contrary to the common acceptation that sugar accumulation in leaves leads to photosynthesis inhibition. Lloyd and Zakhleniuk concluded that “feedback regulation of photosynthetic gene expression is not a major feature of the response of plants to long term accumulation of end-products” [42]. Clearly, this point will deserve further investigation.
By complementation of Arabidopsis suc2 knock-out plants using the AtSUC2 coding sequence under the control of various phloem specific promoters, it was established that AtSUC2 is involved in phloem loading in the minor veins of collection phloem, but also in the retrieval of sucrose in the transport phloem, to maintain high sucrose concentration in sieve elements [42]. The higher proton-motive force in the SE/CC complex compared to surrounding phloem parenchyma cells in the transport phloem of apoplastic species is also in favour of an efficient retrieval mechanism for solutes, especially in apoplasmic loaders [43]. This study also revealed that different cell types, such as companion cells, sieve elements and phloem parenchyma cells, might be competing for resources and that the role of axial sinks along the pathway is not negligible [43].
6 Mechanisms of regulation of the activity of sugar transporters
The activity of sucrose transporters is regulated at multiple levels, including transcriptional, and post-translational ones. In sugar beet [44], it was demonstrated that both sucrose transport activity and accumulation of transcripts for BvSUT1, the phloem sucrose transporter, were negatively regulated by the amount of sucrose. A similar correlation between the amount of transcript, protein and activity was also noted in transgenic potato plants where the expression of StSUT1 gene was inhibited by antisense [45]. Although these findings were in favour of transcriptional regulation, several papers provided evidence for post-translational regulation. A regulation by phosphorylation was first proposed based on indirect evidence [46] and supported by recent reports of a phosphorylation in the plasma membrane of AtSUC1 and AtSUC5 in Arabidopsis [47,48]. However, evidence is still lacking to demonstrate direct regulation by phosphorylation. Since SUC/SUT are proton symporters, their activity is regulated by the proton motive force (membrane potential and pH gradient). This was nicely demonstrated by Carpaneto et al. [49] who showed that ZmSUT1, a sucrose transporter from maize, was able to transport sucrose in the influx or efflux modes when expressed in Xenopus oocytes, depending on the orientation of the proton motive force and the sucrose gradient.
More recently, it was demonstrated that the activity of StSUT1 activity is regulated by the redox potential and its dimerisation status in the plasma membrane [50]. Interestingly, active dimeric forms appeared to be restricted to phloem cells. Phloem sap is a reducing environment and the regulation of StSUT1 activity by the redox potential has been proposed as a way of transducing the oxidative status of the leaves via sucrose [50]. These data point to the fact that sucrose transport activity is tightly regulated and linked to different regulatory networks.
7 Additional power brokers of sugar uptake
Increased sugar export capacity might be required in response to higher carbon assimilation, for example, following transition from a low to high light environment. This is achieved in some species by a remodelling of the cell-wall of companion cells or phloem parenchyma cells, giving rise to the formation of cell-wall outgrowths in transfer cells, which allows an increase of the plasma membrane exchange surface [51]. These modifications potentially lead to an increased number of transporters in this membrane, provided that the transporter density remains constant, which still remains to be proved.
Other membrane proteins are involved in sugar uptake and act on the energetics of transporters, such as the potassium channels and the P type H+/ATPase. Potassium channels, such as AKT2/3 in Arabidopsis, are involved in the regulation of sucrose uptake by regulation of the companion cell-sieve tube membrane potential [52]. Thus, in the Arabidopsis akt2/3 mutant, sucrose content of the phloem sap was only half of the control. Moreover, electrophysiological measurements on protoplasts derived from sieve elements of Vicia faba showed a weak inward-rectifying current reminiscent of the ATK2/3 channel properties [53]. This might be taken as an indirect proof that phloem loading occurs in the sieve element in V. faba stem.
The localization of the H+-ATPase, which generates the proton motive force required for activation of sugar transporters, was located in Arabidopsis in the plasma membrane of companion cells [54,55], consistent with those of sucrose transporter in CC [25]. In the minor veins of V. faba leaves, H+-ATPase was immunolocalized mostly in transfer cells [56]. Because of the complexity of sugar uptake, more thorough investigations on the localization of sugar transporters as well as proteins responsible for the proton motive force, such as ATPase and K+-channels, are clearly needed.
Other sucrose transporters, such as in Arabidopsis AtSUC3 or P. major PmSUC3, were proposed to participate either in sensing, retrieval in transport phloem, or in response to stresses [57,58]. Noteworthy, in grape berry, a hexose transporter was also identified in the plasma membrane of CC/SE interface, suggesting a potential contribution of hexose transporters in retrieval or release [59]. Complete maps of sugar uptake and export are currently constructed and should bring a more general overview of the intricate interplay of sugar transports involved in uptake and export [60]. It was also shown that sucrose, acting as a signal molecule, induces phosphorylation changes of a number of plasma membrane proteins in Arabidopsis, including H+-ATPases and sucrose transporters [47]. This suggests that the concentration of sucrose in the apoplasm might also regulate the proton motive force by phosphorylation changes, therefore potentially participating in the adjustment of its own retrieval or unloading.
8 Polyols and raffinose oligosaccharide species as translocated carbon
Polyols and oligosaccharides of the raffinose family (RFOs) might be abundant in phloem sap [10]. In some cases, their concentration may even exceed those of sucrose in phloem sap [15,19]. The most frequent sugar alcohols to be found are mannitol, found in celery and sorbitol, found in common plantain, apple or peach. In apoplasmic loaders (celery and plantain), their transport recruits a mechanism similar to those of sucrose by sugar proton symporters [61,62]. However, the transport of sugar alcohols is sometimes passive as found in symplasmic loaders in Rosaceae species [15]. The role of polyols as a form of long distance transport of carbon is not clearly understood. Roles of osmoprotectant and radical scavenger were proposed for polyols [63]. In transgenic apple trees modified to produce less sorbitol, sucrose level and enzymes involved in sucrose metabolism were upregulated, so the shoot growth rate was maintained compared to control plants [64]. Therefore, sorbitol may act as a signal molecule, in the apple tree, and the metabolisms and transport of sucrose and sorbitol appear be tightly linked.
The presence in phloem sap of a large amount of raffinose, stachyose and similar oligosaccharides species was associated to the polymer trap model in symplasmic loaders (such as Cucurbita maxima, Coleus blumei, Verbascum phoeniceum), although raffinose is also present in low amount in some apoplasmic loaders, such as Arabidopsis [18]. In cucurbits, raffinose is synthesised in intermediary cells where a galactosyl moiety is transferred from galactinol to a sucrose molecule by the galactinol synthase [65]. Intermediary cell are connected by numerous plasmodesmata to the surrounding cells and sieve elements. Because the size exclusion limit of plasmodesmata connections to sieve tubes is higher, raffinose can only diffuse to the sieve element [66], preventing backward diffusion by a polymer trap mechanism. Interestingly, the galactinol synthase promoter from cucurbits keeps its specificity of expression (intermediary or companion cells in source leaves) also in Arabidopsis and tobacco [65]. The specific inactivation in Verbascum phoenoceum of two phloem galactinol synthase genes inhibits RFO accumulation and dramatically depletes long distance transport of photoassimilates, confirming that the synthesis of RFOs is crucial for phloem transport in such species [67].
9 Non-reducing sugars versus hexoses: no definite rule
It was assumed for long that only non-reducing sugars are transported over long distance in the phloem. Therefore, the presence in phloem sap of reducing sugars, such as glucose and fructose, has been considered as a proof of contamination from surrounding cells. However, this view was challenged recently by the study of van Bel and Hess [68] demonstrating the presence of hexoses in the phloem sap of a number of species from Ranunculaceae and Papaveraceae families. Clearly, this point will deserve further investigation as it may change our view on phloem transport of sugars.
10 Sink regulation of sugar accumulation
Sugars reaching sink cells are used to sustain growth and development or as storage carbohydrates. During the life of a plant, some sinks become predominant, for example, when plants undergo the transition from vegetative to reproductive status. Therefore, different amounts of sugars are allocated to competing sinks according to their status.
Although the first steps of unloading of sucrose are thought to be mainly symplasmic [69,70], the observation that sucrose uptake transporters might be expressed also in release phloem suggest an alternative apoplasmic route for unloading. Indeed, a switch from symplasmic to apoplasmic unloading was observed during fruit formation in grape berry [71]. Conversely, a switch from apoplasmic to symplasmic unloading was observed in the potato tuber during tuberization [72]. In apple fruit, some evidence support a major role of apoplasmic unloading [73]. The pathway for nutrient unloading and the regulation of flux during development was extensively reviewed in legume seeds [74]. Postphloem transport in the seed is complex and involves various pathways, an apoplastic pathway being mandatory at the interface between the maternal and filial tissues. Source activity, phloem path and sink properties are all determinant for the import of nutrients to sinks. However, to what extent phloem loading of sucrose (and thus phloem sap sugar content) is involved in the coupling between source and sink is still a matter of debate [75].
Several attempts were made to increase sink strength, for example by modifying starch synthesis in potato tubers [76] and wheat grain [77]. A spectacular result was obtained in sugarcane where the capacity to store sugar in the stalk could be doubled by expressing a sucrose isomerase [78] in the vacuole of storage cells. Isomaltulose, the product of the isomerase activity is not recognized by plant enzymes and is accumulated in stalk cells at levels comparable to sucrose. Interestingly, this increased storage capacity resulted in an increase in photosynthesis and sucrose transport indicating that these activities may be sink-limited in control plants. This may be explained by the fact that isomaltulose is not a substrate for endogenous enzymes and thus does not trigger the regular metabolic and signalling responses of sucrose or derived sugars. Increased sucrose export capacity was also able to derepress photosynthesis.
11 Changes in sugar allocation in response to stresses
Another response revealing the tight interplay between source and sink demands is the plant ability to allocate biomass to organs required for the uptake of nutrients in case of deprivation. Several studies showed that deficiencies in nitrogen and phosphorus resulted in accumulation of carbohydrate in the leaves, higher allocation of carbon to the roots and higher root to shoot ratio [79]. Increased root surface, mainly through the development of secondary roots and root hairs, was also noted [80,81]. In starved plants, sugars, including sucrose, are required for specific responses. Impairing the delivery of sucrose to the roots by different means (e.g. stem girdling of white lupin [82]) prevented also the induction of genes induced by Pi starvation. Moreover, an increase in local hexose concentration was associated to the induction of root elongation and branching in Arabidopsis [83]. Plants can also allocate a larger part of carbon to the roots when challenged with an herbivory attack, therefore increasing their ability to flower afterwards [84,85]. Phloem transport of sucrose is therefore a key point for regulation of the shoot to root partitioning of assimilates. Impaired sucrose export from leaves leads to starch accumulation and, in those cases, to photosynthesis inhibition [79,86], ultimately reducing plant growth.
12 Other signal metabolites acting at long distance
Despite a considerable interest in trehalose as a signalling molecule [87], no report of trehalose in phloem sap has been made so far. Besides sucrose, many metabolites act as long distance signal molecules in response to nutrient deprivation. Among nutrients, the best described are NO3-, amino acids, glutamate, Pi and glutathione, which were described as signals from shoot to root in response to nitrogen starvation, phosphate starvation or sulphur deprivation [88,89]. Nevertheless, the exact contribution of these various metabolites and how these various signals are integrated remain to be determined. Several phytohormones, such as cytokinin, auxin and abscisic acid, might be involved [88,90], establishing some links between nutrient availability, signalling and control of plant development.
In the setting up of a systemic response to biotic and abiotic stresses, other signal molecules such as methyl salicylate, jasmonate and azelaic acid, a nine-carbon dicarboxylic acid were shown to be translocated a long distance via the phloem [91–93]. These responses may recruit as well other factors, such as sugar, auxin, ethylene and cis-jasmonic acid in a complex network that can yield the most appropriate systemic response to maintain the nutritional, growth and developmental status of the plant [92].
13 Small RNAs: new players along the pathway
Small RNAs including siRNAs and miRNAs were found in phloem sap of various species [6,94,95]. The most abundant class of small RNAs, the short interfering RNAs (siRNA), were first identified in mediating post-transcriptional gene silencing. The siRNA can be derived from endogenous loci, transgenes, or viruses and they are known to be non cell autonomous. A large range of siRNAs, including siRNAs directed against transposons, transgenes or viruses in the case of viral infection, were identified in phloem sap [94,96]. More surprising was the discovery of 3 miRNAs (miR156, miR159, miR167) in phloem sap of pumpkin [94] and the subsequent discovery of more than 32 annotated plant miRNAs belonging to 18 different families identified in phloem sap of Brassica napus [96].
The abundance of such miRNAs was shown to be strongly dependent on nutrition status for sulphate, phosphate or copper [95,96]. During phosphate (Pi) starvation, the induced miRNA399 was shown to move across graft junctions, where it can act on its target, the PHO2 transcripts, in response to nutrient deprivation, confirming a signalling function of the phloem delivered miRNA [96–99]. Although there is a large body of evidence demonstrating that miRNAs involved in patterning or acquisition of cell identity are cell autonomous, the observation that miR399 is transported in the phloem translocation stream from shoot to roots and acts at long distance suggests that at least some stress-induced miRNAs might on occasion act as systemic silencing signals between distant organs [100]. Whether additional miRNAs are also acting long distance on developmental regulatory pathways, such as tuber formation, is under intense investigation. For example, miR172 was for a long time known to play an important role in the induction of flowering in several species [101,102]. Its presence in phloem sap was confirmed in B. napus [96]. Its important role in tuberization in the potato, together with its graft-transmissibility, can be taken as an indirect evidence that miR172 might act as a systemic signal [103].
14 mRNAs: a systemic role in the control of plant development?
Hundreds of mRNAs were found in phloem sap [104–107]. They include mRNAs encoding transcription factors potentially acting on the regulation of meristem activity and development. A role of mRNAs as systemic signal was supported by various observations: (1) the presence in phloem sap of several mRNAs encoding transcription factors from DELLA-like GRAS family, KNOTTED-1-like homeobox and BEL-1-like BEL5 TALE family; (2) their long distance transport through graft unions; and (3) a long distance effect on leaf morphogenesis or tuber formation [108–110]. Consistent with this concept, RNA motifs required for long distance were recently identified on the GAI and the BEL5 mRNAs [111,112].
Likewise, many phloem sap mobile proteins display RNA binding properties. For example, the pumpkin polyuridine binding protein RBP50 [113], the cucurbit PP2 phloem lectin [113] and PP16 [114] displayed mRNA binding activity. The phloem small RNA binding protein 1 (PSRP1) [94] bound selectively to single-stranded small RNA species, whereas the cucurbit PP2 phloem lectin bound viral and viroid RNAs and endogeneous mRNAs [113,115,116]. Several of these proteins were shown to form large complexes [113,117]. Phloem RNA binding proteins, such as RBP50, were shown to be part of large ribonucleoprotein (RNP) complexes, binding mRNA species coding for transcription factors [113]. The authors concluded that the RBP50 system plays a role in the delivery of specific transcripts to distantly located tissues.
However, as pointed out recently [4], the relevance of systemic mRNAs signalling still needs to be clarified, in particular in natural environmental responses, since protein translated from such mRNA might as well be a signal, as shown for the FT protein (flowering locus T) [118,119]. In this case, a first report described the presence of FT transcripts in sieve elements at floral induction [120], but more precise studies definitely established that the FT protein was the long distance signal for the control of flowering time [118]. Noteworthily it was established that not all RNAs present in phloem sap are mobile over long distance, as shown from graft experiments realized in melons [105]. Some of the phloem sap RNAs may not have an essential role, if any, in long distance signalling.
15 Dual functions of sieve element proteins in structure and signalling
A large range of proteins was characterized in phloem sap of monocot and dicot species, which correspond to phloem mobile proteins [107,121–127]. Many of these proteins are involved in the activity and the survival of sieve elements. Frequent classes of proteins corresponded to enzymes from sugar metabolism, antioxidant defence systems, protease inhibitors and proteins believed to act in sieve pore occlusion [121,123–125]. Besides housekeeping functions, several classes or proteins potentially acting on signalling were found and include kinases, calcium binding proteins, RNA-binding proteins and enzymes of the biosynthesis of stress phytohormones [128]. Defence related proteins were also frequently found [127,128]. For a description of membrane proteins of sieve elements or proteins anchored to those membranes, other approaches still need to be developed.
16 Sites of synthesis of sieve tube proteins: an old debate
A few proteins found in phloem sap are synthesised in immature sieve elements and are present throughout the sieve tube life, as demonstrated for the for1/SEO1 component of the forisomes [129], the contractile protein bodies found in legume sieve tubes [130]. However, the vast majority are thought to be synthesized in the companion cells and therefore must be trafficked to SEs via Pore Plasmodesmata Units (PPUs) [131]. This was definitely shown by the observation that the transcription of some genes is restricted to companion cells whereas proteins accumulate both in companion cells and sieve elements, as described for the phloem lectin PP2 in pumpkin or thioredoxin H in rice [132–135].
It is generally assumed that protein synthesis does not take place in differentiated mature sieve elements [2]. This is further supported by cytological observations showing that during the maturation of sieve elements, most ribosomes disappear from the surfaces of the endoplasmic reticulum [136,137]. Surprisingly, in a comprehensive study of pumpkin phloem sap proteome, more than 100 proteins involved in the translational machinery were identified [127], supporting the hypothesis that protein synthesis might occur in enucleate sieve tube systems. These include aminoacyl-tRNA synthetases, ribosomal proteins, translation initiation, elongation, and termination factors. Some of these proteins were found in the phloem sap of rape and rice [125,126], although at that time the authors concluded they might be contaminations from other cell types. These observations are particularly intriguing and need further investigations. Either this machinery is recruited for translation, or, alternatively, it may participate to other cellular processes such as the formation of RNP complexes, as suggested for the translation initiation factor, eIf5A found in at least two phloem sap complexes [113,117].
In a survey of small non coding RNAs present in pumpkin phloem sap, most RNA involved in the translation machinery were also found in the exudates, including 5S, 18S and 26S rRNAs, and full length tRNAs. In this study, aminoacylated tRNAs, which are capable of facilitation of translation, were also found. Nevertheless, the authors observed the presence of truncated tRNAs, presenting inhibitory activity on an in vitro translation assay [138]. This suggested a role in a regulatory mechanism to down-regulate protein synthesis. Alternatively, in response to abiotic stress, tRNA fragments have been shown to generate small RNAs [139], as recently reported in Arabidopsis, in response to phosphate starvation [99]. Altogether, these data support a potential role of tRNAs and tRNA-derived sequences for regulation of expression in the phloem.
17 A role of the ubiquitin-proteasome system
Turnover and continuous synthesis of phloem-specific proteins was demonstrated by strong incorporation of 35S-methionine into phloem-specific proteins collected from phloem exudates [140,141]. The pumpkin proteome survey revealed that phloem sap contains at least 116 distinct components of the proteasome-mediated degradation complex, including ubiquitin-activating, ubiquitin-conjugating and ubiquitin ligase enzymes as well as proteasome subunits, supporting the hypothesis that the functional, enucleate sieve tube system has retained the capacity for proteolysis [127]. These findings were consistent with other reports of ubiquitination components found in phloem sap of rapeseed and rice [125,126]. Interestingly, in plants, the ubiquitin 26S proteasome system targets numerous signalling pathways linked to phytohormones [142], whereas the phloem transports various phytohormones, including auxins, cytokinins, abscisic acid, gibberellins, jasmonates and methylsalicylates [143,144]. Whether the ubiquitin–proteasome system is acting in the sieve tubes for protein degradation or hormone signalling is still an open question.
18 Sieve tube ER and membrane proteins delivery
Many questions are still unanswered: besides soluble proteins, which might traffic through plasmodesmata via the PPUs, how are membrane proteins addressed to their final location within sieve elements? Outstanding insights were brought using an ER-specific fluorochrome and fluorescence redistribution after photobleaching (FRAP) [145]. The authors described an intimate coupling between ER of CC and SE and hypothesised that it may provide a pathway for trafficking membrane proteins into the SE, protected from the rapid stream of solutes passing through the SE lumen. Whether membrane proteins present in the sieve elements are synthesized in companion cells and traffic via the ER to the SE, or whether they are synthesised in the sieve elements remains to be clarified. Examples are the SUT1 sucrose transporter for which mRNAs were found in the SE and in plasmodesmata [20] and the ENOD early nodulin for which their sites of transcription were not determined with precision [146].
19 Role of electrical potential waves and calcium in phloem signalling
The transport of metabolites, phytohormones or macromolecules is thought to be driven by phloem pressure-driven mass flow [147–149]. Its velocity was estimated to range of a few cm.min-1 in the stem of castor bean, poplar and tomato [150]. In Arabidopsis it takes several hours for transport of metabolites from source to sink leaves along an orthostichy [148]. Sieve tubes may also act as low-resistance pathways for systemic electric signals in the form of electrical potential waves (EPWs) [151]. Several classes of EPW were described [151,152], with propagation rates from 5 to 200 cm mn-1, much faster than those driven by chemicals transported by phloem saps. Many examples of responses based on electric signals were reported in plants, such as folding of leaflets in Mimosa pudica in response to cold or touch, arrest of cytoplasmic streaming in the alga Chara, some responses to wounding or burning. In sieve tubes, EPW might trigger systemic induction of protease inhibitors in response to wounding in tomato [153], diminution of phloem sap flow in maize [154], and in V. faba forisome dispersion leading to transitory and reversible occlusion of sieve plates [155].
The transmission of electrical signals within the plant depends on the low electrical conductance of plasmodesmata in the lateral direction, which are scant and mostly closed in transport phloem [43,156] and on the high degree of electrical coupling via the sieve pores in longitudinal direction (Fig. 2) [151]. The transmission of electrical signals along sieve tubes is achieved by ion channels, including Ca2+ channels, K+ channels, Ca2+-dependent Cl- anion channels and plasma membrane H+-ATPase [151,152]. Ca2+ channels were observed by immunodetection on the plasma membrane of sieve tubes in Nicotiana and Pistia [157] and more recently by fluorescently tagged dihydropyridine (DHP) [158]. Resting levels of free cytosolic Ca2+ in sieve elements were measured in V. faba and estimated to 50 nM, consistent with concentrations reported in other plant systems [158]. Much higher concentrations are assumed in close vicinity of SER Ca2+ channels. In response to wounding, Ca2+ can reach higher local concentrations, up to 200 nM. Such elevations in Ca2+ concentrations trigger downstream responses in sieve tubes. In V. faba, the dispersion of forisomes depends upon local high concentrations of Ca2+ delivered from the sieve element reticulum (SER) present at sieve plates and pore plasmodesmata units (PPUs), at threshold levels of more than 40 μM Ca2+ [158]. Therefore, in sieve elements SER would be acting as a reservoir for Ca2+ for local responses.
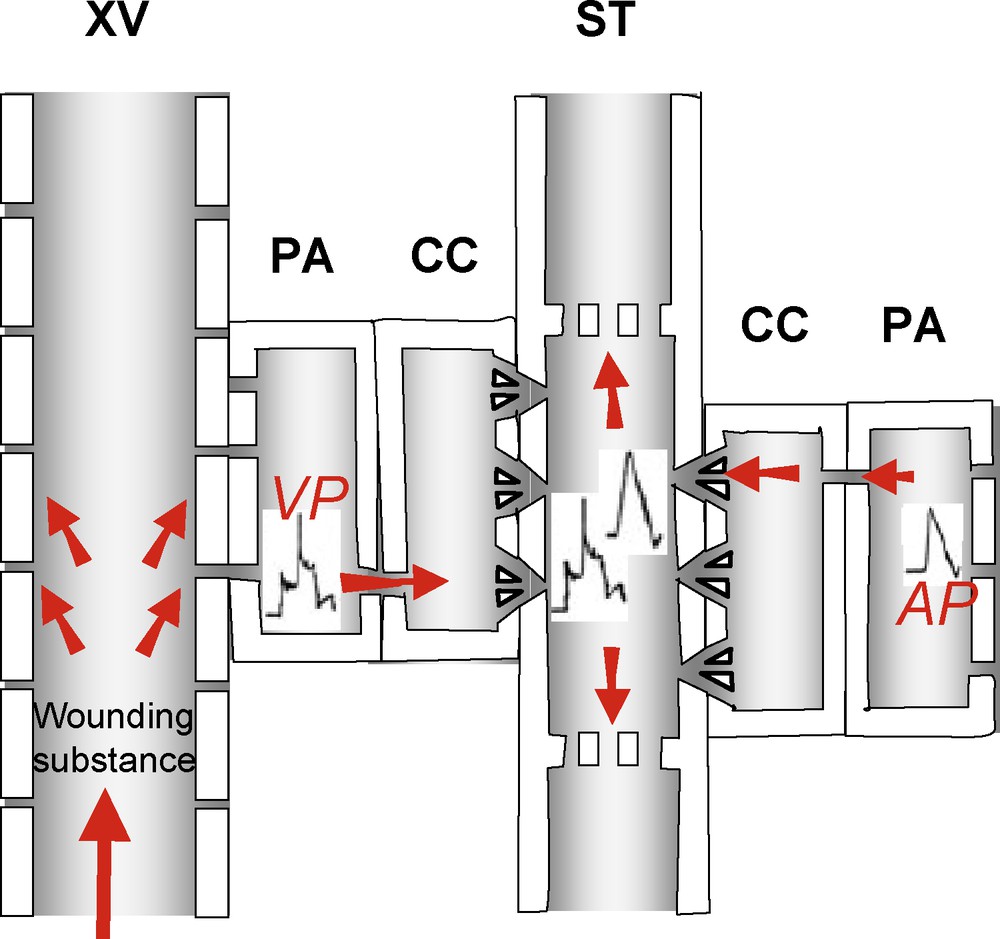
Electrical communication over long distances. Two main electric potential waves are known (modified after Fromm and Lautney, [152]). First, action potential (AP; right) propagates over short distances through plasmodesmata to the SE/CC complex, where it travels over long distances along the SE plasma membrane in both directions. Second, variation potential (VP; left) is generated at the plasma membrane of parenchyma cells (PAs) adjacent to xylem vessels (XVs) by an hydraulic wave or a wounding substance and pass through the plasmodesmal network to reach the phloem, where it can also travel in both directions. PA: parenchyma cells. XV: xylem vessels. ST: sieve tubes.
Intriguingly, a key role played by reactive oxygen species (ROS) in mediating rapid systemic signals in plants was recently described in Arabidopsis plants [159]. It is based on the accumulation of ROS in the extracellular spaces, is independent of stress signalling pathways (mediated by ethylene, JA or SA), but can be triggered by heat, cold, high light or salt stress. The speed propagation of the signal is 8.4 cm.min-1 and this is comparable to the rate observed for system potentials, a novel systemic apoplasmic signal induced by wounding in V. faba and Hordeum vulgare by leaf cutting [152]. Although the contribution of vascular tissues and phloem was not considered in this study, similarities between EPW and some aspects of this new systemic signalling pathway may suggest an implication of apoplasm of the phloem for transmission of the signal mediated by ROS.
20 Concluding remarks
For many years, phloem has been regarded as a conduit for the translocation of photoassimilates. The presence of non-reducing sugars, such as sucrose, polyols, raffinose and stachyose was referred to as a hallmark of phloem sap in which reducing sugars such as glucose and fructose were not present. Consistent with this view, an overall picture of the mechanisms of sucrose loading emerged for apoplasmic loaders, believed to be similar for loading of sugar alcohols. This was completed by the description of polymer trap mechanism acting on the diffusion of RFOs in symplasmic species, such as Cucurbitaceae or V. phoeniceum [67,160]. However, other loading strategies exist, and might eventually coexist, especially in woody species in which passive loading appear to be common, for the transport of sucrose and sugar alcohols [14,161].
Still, several additional features are currently reconsidered. First, large amounts of hexoses were found in phloem sap of Ranunculaceae and Papaveraceae [68], and the authors proposed that their presence might be found in other herbaceaous species. They urged a rethinking of the views of phloem carbohydrate transport, which could be for example a re-examination of the role of hexose transporters or other enzymatic activities in sink tissues. Second, components of the translation machinery were found in the phloem sap albeit direct synthesis of proteins in sap extracts has yet to be tested [127]. Since ribosomes cannot be observed in sieve elements using transmission electron microscopy imaging, this result remains intriguing, and prompts us to wonder whether such translation factors might be recruited for other mRNA related functions in sieve elements. Finally, a major role of the transport phloem, especially in retrieval of sugars, was established by several approaches [40,43].
New issues recently came under the spotlight. The phloem is a reducing environment in which low oxygen tension favours the degradation of sucrose by SuSy and UGPase to save energy and oxygen and the subsequent limitation of glycolysis and respiration [162]. With such a cell energy status, an emerging role of redox potential was identified in the control of sugar transport [49], providing a new link between redox signalling and sucrose signalling in the phloem. In addition to sucrose, many more signal molecules were identified in phloem sap, including metabolites, hormones, proteins, siRNA, miRNA and mRNAs and secondary messengers. These contribute to signalling either cell to cell from different cell types within the phloem (phloem parenchyma cells, companion cells and sieve elements) or long distance in sieve tubes between various source and sink organs. Such molecules are transported by mass flow, provided that they are not diverted along the phloem path. Even so, the molecular mechanisms controlling the delivery of macromolecules and metabolites along that path and between various sinks are poorly understood.
Faster moving signals, in the form of electrical depolarisation, also take place within sieve elements [151,152] and recently the propagation of a redox wave with intermediary speed was described [159]. Therefore, signal transmission in the phloem could occur at different speeds and trigger modifications of different targets: whereas electrical depolarisation will affect very rapidly the activity of channels and transporters and move along the vascular system, the effects of small RNAs, mRNAs or proteins on their target will take longer. Hence, phloem is a central network integrating numerous signalling pathways (Fig. 3), transmitting a large range of signals while supplying energy and nutrients to competing sink organs and ultimately regulating major aspects of plant growth and development.
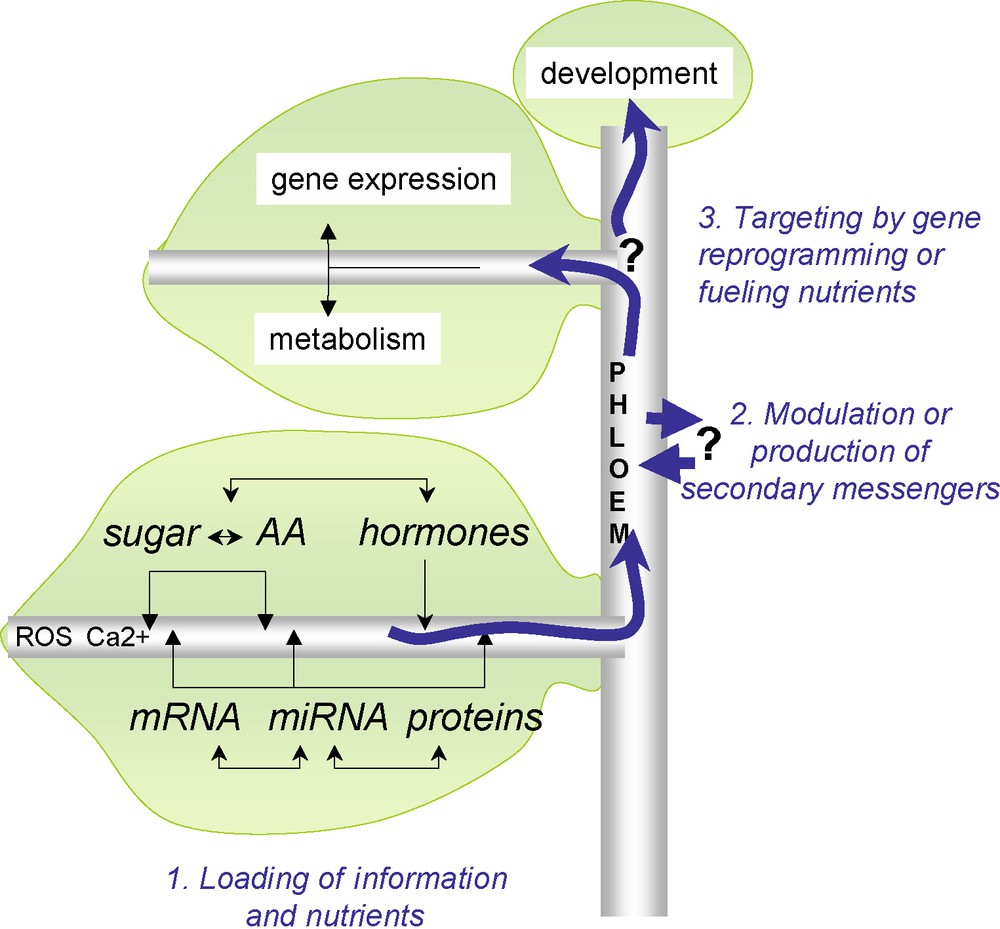
Schematic representation of signalling pathways and nutrients trafficking into the phloem. In source photosynthetic leaves, metabolites (sugars and amino acids), hormones, macromolecules and other signals (ROS, Ca2+ etc.) are loaded into sieve elements. This depends on symplasmic transport via plasmodesmata and on apoplasmic transport via transporters. The redox potential is involved in the regulation of some transporters and might affect the loading of other molecules. An important step is carried out in the transport phloem for retrieval of molecules along the path. The delivery of these signals and nutrients will enable gene expression reprogramming (miRNA, proteins, sugars, etc.), will affect the metabolism of sink organs and subsequently will act on the development of newly developed organs.