1 Introduction: ‘Genetics’
In a letter to his colleague Adam Sedgwick in 1905, the English biologist William Bateson (1861–1926) used the word ‘genetics’ to designate ‘the science of heredity and variation’. Bateson was then known as one of the major Mendelians in the world, and proposed using the word ‘genetics’ to name the chair that was created for him at Cambridge in 1906. In the end, Bateson's chair was named ‘chair of biology’, but on the occasion of the third International Conference on Plant hybridization, Bateson proposed that the new science of heredity based on Mendel's laws be named ‘genetics’. This proposal was enthusiastically approved and the 1906 Conference was published in 1907 as ‘Report of the Third International Conference 1906 on Genetics’. This periodical meeting still exists. In spite of deep theoretical changes, some of which are described hereafter, the scientific discipline of genetics has maintained itself.
2 Origins of genetics: from Mendel to Mendelism
When was genetics born? Was it in 1866, year of the publication of Mendel's memoir on plant hybridization [1]? Or in 1900, when three botanists, Hugo de Vries in the Netherlands, Carl Correns in Germany, and Erich von Tschermak in Austria, independently rediscovered Mendel's laws? Or in 1902 when Bateson's book, A Defence of Mendel's Principles of Heredity explicitly connected Mendel's laws with the general question of ‘heredity’ [2]? Or in 1906, when Bateson first made public use of the word with reference to Mendel? There cannot be a definitive answer to this question. Mendel's experimental work on peas was crucial, but only in a methodological sense. Mendel's intention was not to offer general laws of heredity, but only a ‘law of the development of hybrids’ in plants; furthermore, Mendel's memoir remained largely unknown until 1900, when his ‘laws’ (plural instead of singular) were rediscovered. This rediscovery would also be an ambiguous date of birth for genetics, because those who rediscovered it did not intend to propose general laws of heredity either, but only of hybridization. Bateson's 1902 book was certainly a key event, because it showed that Mendel's first law (the law of segregation, applying to just one character) applied not only to plants but also to animals; Bateson also defended that the Mendelian laws of hybridization did not apply only to the results of crosses between individuals of distinct varieties or species, but to a huge number of individual hereditary differences among virtually all sexually reproducing organisms. This book also introduced a technical vocabulary that rapidly became indispensable for all Mendelians: ‘allelomorph’ (or, more simply, ‘allele’), ‘homozygote’, and ‘heterozygote’; these terms imply that for a given character transmitted in a Mendelian way, each individual has two (and exactly two) physical versions of the same hereditary element — an idea that Mendel did not suggest (Fig. 1). Finally, 1906 would be too late a birth date, because a significant international community of Mendelians already existed by then. What occurred in 1906 was the official creation of ‘genetics’ as a discipline in the institutional sense, with a name, a clearly international network, and an international meeting devoted to it. But this was of course the result of a complex intellectual history that cannot be given here in detail [3].
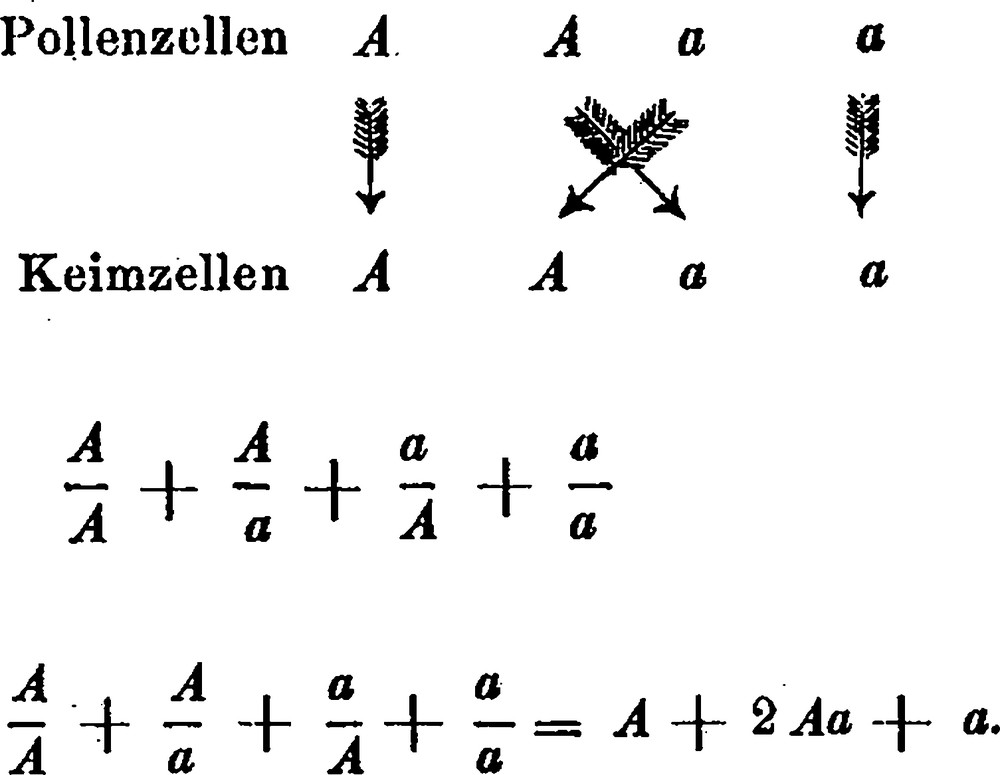
Three formulae used by Mendel in his 1866 memoir for explaining the ratio observed of one character ([1], p. 30). The two parents belong to, respectively, type A and type a (for instance yellow and green peas. A is dominant over a. The first formula represents what happens during fertilization: pollen cells (pollenzellen) associate with ovarian cells (Keimzellen). The four combinations represented are equiprobable. The second figure represents the result in the zygote (numerator: male origin; denominator: female origin). The third formula shows the proportions of three types in the progeny: two pure parental forms (A and a), and one hybrid form Aa. If crossed between them, these Aa will give again a mixture of pure and hybrid progeny. The second formula shows how close Mendel was to the spirit of genetics. But the third formula shows that he did not have the notions of genotype and allele. In Mendelian genetics (here distinguished from Mendel), the second member of the equation would be: AA + 2Aa + aa.
Two additional conceptual and linguistic events should be added to make the Mendelian phase of the history of genetics clearer. One is Hugo de Vries’ use of the words ‘pangenesis’ and ‘pangene’ in a book published in 1889 as Intracellular Pangenesis [4,5]. In this book, De Vries supported the existence of hereditary particles in all the cells of an organism. For these particles, he coined the word ‘pangene’, a word inspired by Darwin's ‘pangenesis’, although De Vries’ pangenesis rejected the Lamarckian part of Darwin's hypothesis, namely the conjecture that all the cells of an organism propagate little pieces of their cytoplasm (‘gemmules’) that circulate through the body and are finally kept in the germinal cells. The term ‘pangene’ is the origin of Wilhelm Johannsen's ‘gene’, proposed in 1909 in the important book where he also introduced the words ‘genotype’ and ‘phenotype’ [6]. Johannsen was responsible for the standard meaning of the ‘term’ gene that dominated until the emergence of the molecular concept of the gene: no more than a ‘calculating unit’ intervening in Mendelian crosses, with no morphological hypothesis about the nature of the Mendelian determinants.
3 Incorporation of knowledge on chromosomes into genetics: classical genetics, 1915–1950
In the two last decades of the 19th century, the morphology of chromosomes and the processes of mitosis and meiosis began to be relatively well known. Following August Weisman, some leading cytologists suggested that the remarkable behavior of chromosomes during cell division was important for the knowledge of variation and heredity. As early as 1902, Walter Sutton and Theodor Boveri proposed to consider the chromosomes as the bearers of the Mendelian factors. They rightly thought that the process of meiosis, that is to say the two successive cell divisions leading from a diploid cell to the four haploid cells that generate the gametes, was the basis for Mendel's laws of segregation and reassortment (mixing of Mendelian factors into new combinations). Some Mendelians quickly adopted this conception, but for a number of them the ‘chromosomal theory of heredity’ remained an erroneous theory for more than a decade. For instance, William Bateson, in a sense the founding father of genetics, never accepted this theory. Conversely, Thomas Hunt Morgan (1866–1945), who was the main architect of the fusion between Mendelian genetics and the chromosomal theory, did not accept the former in the early 1900s. In 1908, however, he began working on heredity with Drosophila melanogaster (fruit fly), using both the chromosomal theory of heredity and Mendelian genetics. In 1915 this led him to publish, with three other colleagues, all of whom became major geneticists, what is probably the most important book in the entire history of genetics, The Mechanism of Mendelian Heredity [7]. This book was translated into French in Brussels in 1923 [7].
The fusion between the chromosomal and the Mendelian theories had many remarkable effects. If Mendelian factors or genes were part of the chromosomes, then it was easily understandable why two copies of every gene exist in all the cells of a diploid organism. This provided a mechanistic foundation for Mendel's first law, by which a zygote receives only one version of a given gene from each parent (law of segregation’, also called ‘law of the purity of gametes’). But the chromosomal theory also explained why Mendel's second law (the law of the reassortment of genes) has many exceptions, since this law does not apply when two genes located on the same chromosome segregate together. Moreover, the fact that homologous chromosomes are able to make chiasmata and to exchange strands with each other also explained why genes could recombine in spite of being located on the same chromosome. Morgan called this phenomenon ‘crossing-over’ (Fig. 2).
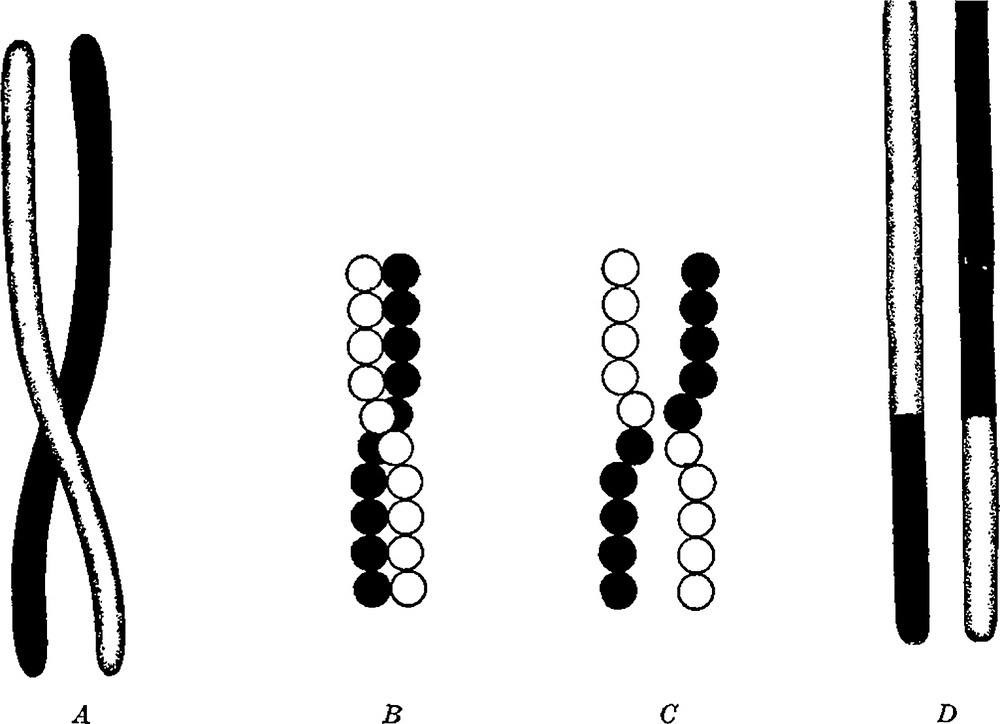
The diagram used by Morgan et al. to represent crossing over (Fig. 24 in [7]. Comment of the authors: ‘At the level where the black and the white rod cross in A, they fuse and unite as shown in D. The details of the crossing over are shown in B and C.’ This figure illustrates the convergence of the Mendelian way of thinking and the chromosomal (or cytological) way of thinking. Chromosomes are interpreted as a sequence of genes.
In the 1920s, the chromosomal theory had become an essential part of genetics and it gave a more material flavor to genetics. In the new theoretical framework, the genes had spatial significance: they were located on chromosomes, and they occupied a precise location each relative to the others: their ‘genetic distance’ could be calculated on the basis of the proportion of crossing overs. In addition, in particular cases (e.g., the giant polytene chromosomes found in the salivary glands of Drosophila), the genetic distances could be compared with the physical irregularities directly observable on the chromosomes with the help of a microscope. The chromosomal theory also permitted a relatively precise meaning to be given to the notion of gene mutation. After the pioneering work of Herman Muller in the 1920s on the effect of X-rays on Drosophila, a genetic mutation was defined as a local alteration of a chromosome: a particular allele was transformed into another one, the ‘mutant gene’. Nevertheless, before the advent of molecular biology, geneticists hardly knew what the material nature of the genes was: were they parts of molecules, or entire molecules, or aggregates of molecules, or subcellular organelles, or recurrent physiological cycles? Furthermore, their physiological mode of action remained enigmatic.
An interesting effect of the chromosomal reinterpretation of genetics was to pluralize the operational characterization of the gene as a ‘hereditary unit’. In the original and strictly Mendelian perspective, a gene was no more than a unit of function: something transmitted in a discrete manner, and the substitution of which has a functional effect observable in the phenotype. In the context of chromosomal genetics, a gene is also a unit of recombination (intra-chromosomal recombination resulting from crossing-overs). And finally, Muller's X-ray induced mutagenesis experiments introduced the notion that a gene is a unit of mutation. Remarkably, these three notions coexisted harmoniously (or almost) until the discovery that genes are made of DNA.
4 Institutionalization of genetics
The role of the international meetings on hybridization, which became the ‘International Meetings of Genetics’ has been already mentioned. Other signs of the institutionalization of genetics in the early 20th century include: the creation of chairs explicitly devoted to genetics (e.g., Punnett, UK, 1912; Baur, Germany, 1913; Serebrovsky, USSR, 1930), and innumerable courses of genetics all over the world; the creation of specialized journals (e.g., Journal of Genetics, 1910; Hereditas, 1920); —the publication of textbooks and treatises exclusively devoted to genetics (18 in English and 7 in German between 1902 and 1918, none in French [8]). In addition to these institutional aspects, economic stakes were tremendously important in fostering the development of the new science: animal breeding, plant breeding and horticulture were powerful incentives, and provided resources for genetic research in all advanced countries, including France, where the Vilmorin Company sponsored and hosted the 4th International Meeting of Genetics in 1911.
Institutionalization also meant specialization. In the mid-1930s, genetics was conventionally subdivided into three major sub-disciplines: formal genetics proper; population genetics, which provided the main theoretical basis for the Modern Synthesis; and physiological genetics, the aim of which is to study how the genes produce their effects (or, in modern terms, the mechanisms governing the expression of genes).
5 The emergence of molecular genetics
A decisive step for the connection of genetics with biochemistry was Beadle and Tatum's 1941 paper ‘Genetic control of biochemical reactions in Neurospora’, which offered the first proof that a specific gene controls a biochemical reaction (namely the production of vitamin B6). In this seminal paper, they proposed that ‘genes control or regulate specific reactions in the system either by acting directly as enzymes or by determining the specificities of enzymes’ [9]. In the following years, still working on the mold Neurospora crassa, they showed that a single gene controls each step in a metabolic pathway. This led to the famous ‘one gene–one enzyme hypothesis’ [10]. However, in the late 1940s, the molecular nature of the genes still remained unknown. Most biologists, including Beadle and Tatum, thought that genes were proteins, because proteins are complex macromolecules with remarkable catalytic properties. Relying on this common belief, the physicist Erwin Schrödinger proposed a striking characterization of the gene in his 1944 book, What is Life [11]. According to Schrödinger, a gene is an aperiodic crystal with exceptional properties, since this molecule is both hetero-catalytic (i.e. like an ordinary enzyme, it catalyzes a metabolic reaction), and auto-catalytic (the gene catalyzes the reaction that enables its own replication).
In such a context, the remarkable experiment conducted by Avery, MacLeod, and McCarty in 1944 [12] came as a surprise. This experiment showed that purified DNA extracted from a dead virulent pneumococcus was able to ‘transform’ a non-virulent strain of pneumococcus (a bacterium able to cause acute pneumonia) into a virulent strain. But it was only after Francis Crick and James Watson's discovery in 1953 of the structure of the DNA molecule that DNA became the molecule that carries the hereditary properties. This was the beginning of an exceptional succession of discoveries in molecular biology in the 1950s and 1960s, among which the discovery of the genetic code and the first model of regulation of gene expression by François Jacob and Jacques Monod were particularly important. We will not detail this extraordinary harvest of new biological knowledge here (for more on this subject, see [13]). The rest of this paper will concentrate on the effects of molecular biology on the concept of the gene, which has been the central concept of genetics for more than a century.
6 From the classical to the molecular concept of the gene and beyond
The works of Seymour Benzer (1921–2007) on the Bacteriophage T4, a virus infecting bacteria, are an exceptional theoretical event in the history of genetics [14]. Realized in the mid-1950s and early 1960s, they were probably the ultimate attempt to build a rigorous genetic concept of the gene, although they were conducted in an experimental context already focused on genes as sequences of nucleotides. Benzer showed that recombination (crossing over) can occur in many places within a single gene, and this allowed him to discover the fine molecular structure of the gene. Simultaneously, Benzer showed that mutation events could also affect a given gene at many sites. This was the origin of the notion of ‘punctual mutation’, that is to say a mutation consisting in substituting a single nucleotide with another one. Benzer was quick to conclude that his experiments meant the dissolution of the traditional characterization of the gene as being simultaneously a unit of function, a unit of recombination, and a unit of mutation. In the new molecular context, the typical mutation unit is the nucleotide; the recombination unit consists of two adjacent nucleotides; and the functional unit is the sequence of nucleotides able to perform a physiological function (e.g., controlling the production of a protein). In the course of his mapping of the chromosome sequence of the T4 virus, Benzer massively used the ‘cis–trans’ test, a test that permits to distinguish the mutations that affect different genes from different mutations affecting the same gene. This test is exclusively based upon genetic methods, that is to say on the examination of the result of crosses. Benzer proposed to adopt it as the basis for the definition of the new concept of the gene that he proposed, in replacement of the obsolescent ‘classical concept’, the ‘cistron’ concept. A cistron is a unit of genetic function, identified by applying a cis-trans test. This genetic concept of the gene proved immensely useful to a number of molecular biologists, in particular François Jacob and Jacques Monod, who used it abundantly in the experiments that led them to the discovery of the lactose operon, which offered the first effective model of how the expression of genes is controlled. This definition of the gene is still in use today, especially among those who are not satisfied with the molecular definitions of the gene that have been proposed since the 1960s.
Nevertheless, for approximately 50 years, a molecular definition of the gene has prevailed. The original idea, proposed triumphantly around 1960, was that a gene is no more nor less than a DNA sequence that codes for the amino-acid sequence of a protein (or, more generally, a polypeptide). However, as early as the mid-sixties, the original molecular definition had to be softened. Should the name ‘gene’ be restricted to the coding sequence of a structural gene, or should the sequences involved in regulation (repressor gene, promotor and operator sites) also be accepted as ‘genes’? And what about the DNA sequences determining the RNA sequence of ribosomal units, or of transfer RNAs? Moreover, in the late 1960s it was known, as in the case of the lactose operon discovered by Jacob and Monod [15], that some of these entities overlap, e.g., the promotor site (the sequence where the RNA polymerase that ‘reads’ the DNA sequence and makes RNA sequence binds) and the operator site (the sequence that receives the repressor protein), or—even worse—the operator sequence and the first structural gene. Such findings showed that it was difficult to provide a non-ambiguous and general molecular definition of the gene. And it was also impossible to offer a molecular equivalent of the definition of the gene given by classical genetics. In 2000, at a conference celebrating the 100th anniversary of the rediscovery of Mendel's laws, the author of the present paper asked François Jacob whether he and other molecular biologists were aware of how much the very notion of gene was threatened by his findings. François Jacob answered: ‘Yes, we were aware of these theoretical difficulties, but we chose not to speak too much of them; the priority was to move forward.’
7 New challenges for the molecular concept of the gene
The invention of recombining DNA technologies (commonly called ‘genetic engineering’) in the late 1970s has led to an impressive list of discoveries, which have rendered the hope of finding a non ambiguous molecular definition of the gene more and more unlikely. These discoveries include the discovery of split genes, which was certainly what first led molecular biologists to recognize the limits of the molecular definition of the gene as a ‘coding sequence’. Split genes, commonly found in eukaryotes and viruses, are genes composed of a succession of exons (some of which corresponding in part or in totality to coding sequences) and introns (or intervening sequences eliminated after splicing of the exons at the RNA level). As early as 1979, Francis Crick recognized openly that this phenomenon rendered the current molecular concept of the gene a problematic one. In a famous review on ‘split genes’, he ironically wrote in the one and only footnote that comes at the end of the article: ‘throughout this article I have deliberately used the word ‘gene’ in a loose sense since at this time any precise definition would be premature’ [16]. A few years later, the situation became even worse with the discovery of alternative splicing. In alternative splicing, a given ‘split gene’ can code for various different proteins, depending on whether this or that exon is expressed at a given time (for instance a certain exon is expressed in embryos, another one is expressed in the adult organism). Other phenomena are also quite challenging for the notion of a gene as ‘no more than a coding sequence’: assembled genes (where germinal sequences, often designated as ‘genes’, are assembled to make a single somatic gene, a situation commonly found in immunogenetics: all antibodies are coded by assembled genes); inversion of the reading frame (meaning that the same DNA sequence can be transcribed in both directions, resulting in different proteins); partial overlapping of the reading frames (the same sequence translated in different frames can give up to two or even three different proteins); multiple initiation and termination sites of transcription (producing a multiplicity of RNA molecules out of which proteins will eventually be synthesized); non-universality of the genetic code (e.g., a slightly different code for nuclear genes and cytoplasmic genes: this means that the same sequence, in the same organism, can lead to different proteins). This is only a partial list. Today, many molecular processes are known that challenge the traditional ‘one gene-one protein’ dogma. In reality, it seems hopeless to provide a general definition of the gene on the basis of exclusively molecular criteria (for a comprehensive view, see [18,19]).
The discovery of non-coding RNA has maybe been the most impressive discovery in molecular biology since 2000. Recent data show that 98.5% of our genome is not translated into proteins, but more than 70% is transcribed into RNA. Furthermore, 70,000 promoter regions (the sites where proteins bind to control gene expression) and 400,000 enhancers (regulatory sites that affect the expression of distant genes) have been discovered in the human genome [17]. These findings suggest that the information contained in our genome goes far beyond the usual picture of 20,000–25,000 protein-coding genes. There are many more functional units than those protein-coding genes. Given this situation, one might think that the word ‘gene’ could be abandoned, and replaced by more precise terms. This is what an increasing number of molecular biologists are choosing to do in practice. But the real situation for biology as a whole is more complex. Genetics is not only a concern for molecular biologists, but also for a certain number of disciplines where the concept of the gene and other related traditional genetic concepts remain important: evolution (population genetics), behavioral ecology, medical genetics, would be dramatically handicapped if they were deprived of these concepts.
Finally, epigenetic phenomena should also be mentioned. In its modern sense, the word ‘epigenetics’ refers to the study of modifications that directly affect the expression of genes, but are not reducible to changes in the DNA sequence. Current examples are the methylation of nucleotides (i.e. fixation of a methyl radical on a nucleotide), and changes in the configuration of histones (proteins closely associated to DNA in the chromosomes of eukaryotes; they play a decisive role in its compaction). In both cases, these changes alter the expression of genes. The common feature of these changes is their stability, but this stability is more or less pronounced: some epigenetic modifications are transmissible from one generation to another while others are not. Some patterns of methylation seem to be transmissible, and therefore function as alleles, from a genetic point of view. Histone modifications are not transmissible. In reality, there is no consensus about the definition of epigenetics. This is why the ‘Epigenomics Mapping Consortium’ of NIH (National Institutes of Health, USA) recently defined epigenetics in order to give room to both heritable and non-heritable changes: ‘For purposes of this program, epigenetics refers to both heritable changes in gene activity and expression (in the progeny of cells or of individuals) and also stable, long-term alterations in the transcriptional potential of a cell that are not necessarily heritable’ [20].
8 Conclusion
The findings of molecular biology have deeply altered the theoretical framework of genetics. Some authors claim that genetics has been ‘replaced’ by the concepts and methods of molecular biology, the object of which incidentally is not only to study inheritance. For these authors, the ambiguities associated with concepts such as ‘gene’, ‘allele’, ‘locus’ are no more than traces of a past now closed. This is probably too extreme a position. As often in the history of science, theoretical frameworks do not totally replace one another, but partially overlap. For instance, relativist and quantum mechanics have not abolished classical mechanics. Classical mechanics still remains a useful tool for explaining, predicting, and acting for a huge number of phenomena in physics. Similarly, in biology, genetics remains indispensable at certain level of description, especially when heredity rather than physiological functioning is the key problem: evolutionary biology and medical genetics are obvious examples of this situation. Therefore, rather than saying that the concept of gene—and therefore genetics—is dead [21], we prefer to conclude that its relevance is a matter of scientific context.
Disclosure of interest
The author declares that he has no competing interest.
Acknowledgements
I thank Bernard Dujon for his attentive reading of the paper and for his useful suggestions regarding sections 5 and 6 devoted to molecular biology. I am also indebted to Jean-René Huynh for a fruitful discussion on section 6. Véronique Charrière is warmly thanked for her linguistic corrections and suggestions.