1 Introduction
A molecular network in the crystalline phase is defined as a supramolecular structure composed in principle of an infinite number of molecular building blocks or tectons 〚1〛, i.e. informed molecular components containing in their backbones recognition sites disposed in a specific manner and thus capable of mutual specific interactions. The construction of large molecular networks (10–6–10–3 m scale) in the crystalline phase with predicted and programmed structure may hardly be envisaged through step-by-step-type strategy. However, the preparation of such highly ordered solid materials may be reached through iterative self-assembling processes 〚2–4〛 between tectons containing within their structure recognition sites and a specific assembling algorithm that will operate when complementary tectons are allowed to interact. This strategy called molecular tectonics 〚5〛 is a viable and efficient approach and frequently used by nature in both biological and mineral worlds. The molecular tectonic approach relies on a double analysis: (i) at the molecular level, dealing with the individual tectons composing the solid, and (ii) at the supramolecular level, dealing with the inter-tecton interactions 〚6,7〛. The design of specific tectons leading to predicted molecular networks in the crystalline phase still remains a challenge. Concerning the design principles, in order to achieve an iterative assembling process, the tecton must fulfil both structural and energy criteria. In particular, the complementary tectons must, on one hand recognise each other and thus generate the assembling core, and on the other hand must allow the translation of the recognition patterns which lead to the formation of 1-D (a single translation), 2-D (two translations into two distinct space directions) or 3-D (three translations into three distinct space directions) network. In terms of interaction energy between tectons at the level of the assembling core, one may use any types of reversible bonding processes. Hydrogen 〚8–11〛 and coordination 〚12–16〛 bonds have been almost exclusively used for the formation of molecular networks. We have proposed to use mainly rather weak van der Waals interactions for the design of inclusion networks in the crystalline phase 〚17–21〛. As mentioned above, recognising a network in a molecular crystal is a matter of translation of the recognition pattern. As long as the energy of interaction defining the assembling core is larger as in the case of strong H-bonds (charge assisted or not) or substantially greater, as in the case of coordination bonds than other interactions responsible for the formation of the crystal (three dimensional compaction of the individual networks), the network may be defined without ambiguity. However, for inclusion networks excessively based on van der Waals interactions, the task is not obvious, since the packing of the components may also be ensured by the same type of interactions. This difficulty may be overcome by considering the recognition pattern, formed by inclusion of a section of the connector by the cavity of the koiland, as a supramolecular synthon 〚22〛. The term supramolecular synthon here means a recognition pattern that by translation would become a structural node of the network.
2 Results and discussion
2.1 Design and synthesis of koiland
The chemistry of inclusion complexes based on host and guest molecules, i.e. the inclusion of a substrate within the cavity of a receptor molecule, is an established area 〚23–25〛. The basic concept for the design of inclusion networks (koilates) is based on an inclusion processes between concave and convex molecular objects. Thus, the design of inclusion networks is based on the use of koilands 〚17–21〛, which are multicavity receptor molecules composed of at least two cavities arranged in a divergent fashion (concave tectons) and connectors (convex tectons) capable of being included within the cavities of the koiland. The interconnection between consecutive koilands and connectors through inclusion processes leads to non-covalently assembled polymeric species called koilates or inclusion networks. For example, linear koilates (one-dimensional linear molecular arrays) were obtained in the solid state using van der Waals interactions between koilands possessing two divergent cavities with an angle of 180° between them, and linear connectors possessing two extremities, each one capable of being included within the cavities of the direceptor (Fig. 1a) 〚26–31〛.

Schematic representation of a 1-D inclusion network (koilate) by interconnection of consecutive koilands by connector molecules (a), of two possible self-inclusion 1-D koilate formed by self-inclusion of consecutive koilands (b), of a 2-D koilate formed by self-inclusion of consecutive koilands (c) and of the formation of a discrete exo-binuclear complex formed in the presence of molecules acting as stoppers (d).
In terms of design of koilates, one may also explore other possibilities based on self complementary koilands possessing both a concave moieties acting as receptors and convex parts acting as connectors. In that case, the 1- and 2-D inclusion networks may be formed by self-inclusion processes (Fig. 1b and c).
In the present contribution, we report the design and structural characterisation of both 1-D and 2-D inclusion networks based on the self-complementary koiland 2. The latter possessing two pre-organised cavities as well as ethyl groups at both faces of the molecule, which may act as connector parts fulfil the above-mentioned requirements.
We have demonstrated that calix〚4〛arene derivatives (Fig. 2) are candidates of choice for the design of koilands 〚19–21〛, since they offer a pre-organised and tuneable hydrophobic pocket surrounded by aryl moieties as well as four hydroxy groups for further functionalisation. Calix〚4〛arenes bearing four free OH groups or O-alkylated derivatives with short alkyl chains present four limit conformations (cone (c), partial cone (pc), 1,2-alternate (1,2-a) and 1,3-alternate (1,3-a)). Among all conformers, only the cone may be used for the design of koilands. Furthermore, in terms of flexibility of design, calix〚4〛arene derivatives, easily prepared in large quantities, present a further advantage, which is due to the possibility of controlling both the entrance and the depth of the pre-organised cavity by the nature of the R substituents at the para position.
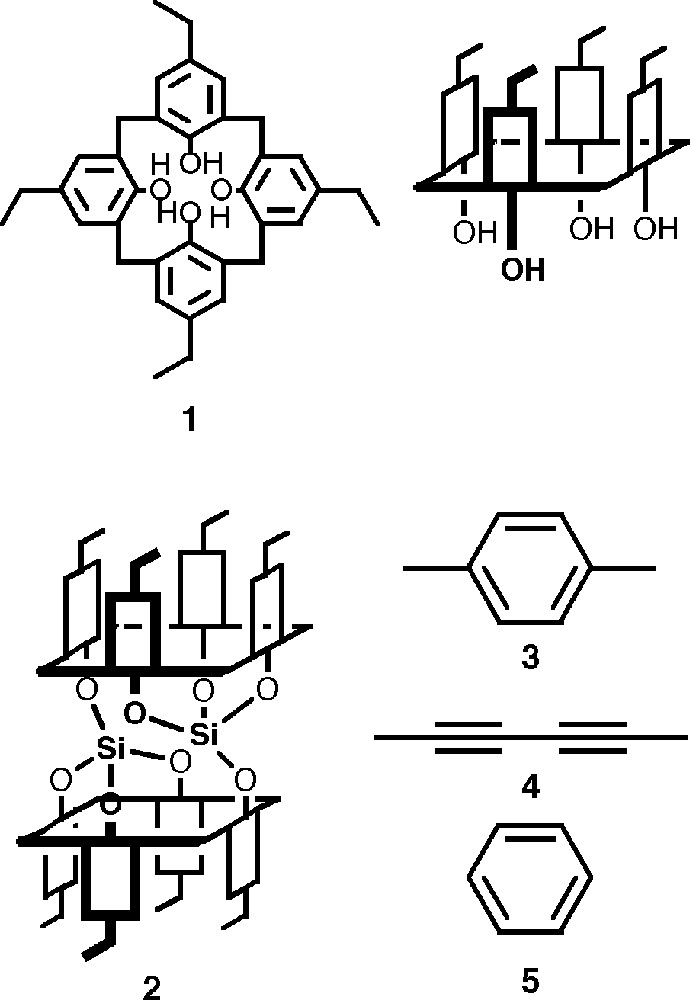
Structures of p-ethylcalix〚4〛arene 1 (top left) and schematic representation of its cone conformation (top right), schematic representation of the koiland 2 obtained upon double fusion of two calix units 1 by two Si atoms and connector molecules used 3 (p-xylene), 4 (2,4-hexadiyne) and 5 (benzene).
Taking into account that a calix〚4〛arene moiety offers four OH groups, two of such a molecule in cone conformation and in a face-to-face-type arrangement would present eight convergent hydroxy groups. Thus, the fusion of two calix〚4〛arene derivatives in the cone conformation may be achieved by two centres adopting tetrahedral coordination geometry such as silicon atom (Fig. 2) 〚17–19〛.
The choice the koiland 2, based on double fusion of two p-ethylcalix〚4〛arene units 1 by two Si atoms, was based on our previous observations on other koilands composed of two p-tert-butylcalix〚4〛arenes 〚17〛 or p-i-propylcalix〚4〛arenes 〚30〛. Indeed, due to the bulkiness of the tert-butyl and i-propyl groups, although inclusion networks could be formed in the presence of appropriate connectors in both cases, no network based on self-inclusion phenomena could be obtained. On the other hand, when using the koiland based on the double fusion of two p-allylcalix〚4〛arenes by two Si atoms, a self-inclusion network was observed in the absence of any connector in the solid state 〚32〛. Thus, we thought that by reducing the steric demand of the alkyl fragment, from tert-butyl to ethyl, at the upper rim of the calix unit, we could obtain self-inclusion networks.
The synthesis of 2 was achieved in 30% yield upon treatment of 1, prepared according to published procedures 〚33,34〛 by NaH in dry THF followed by addition of SiCl4. Compound 2 was obtained as a white solid after chromatography on silica. In addition to 1H and 13C NMR spectroscopy and to elemental analysis, compound 2 was also characterised by 29Si NMR in CDCl3, which revealed a unique signal at – 112.66 ppm as expected.
2.2 Formation of discrete binuclear inclusion complex
In order to study the inclusion ability of 2, the latter was crystallised from solvent mixtures such as p-xylene 3, 2,4-hexadiyne 4 in CHCl3 and benzene 5. In the presence of p-xylene 3, compound 2 formed a discrete exobinuclear inclusion complex in the solid state (Fig. 1d). Single crystals were obtained upon slow diffusion of isopropanol into a p-xylene 3 solution of 2. X-ray analysis (Fig. 3) revealed that compound 2, possessing a centre of symmetry, was composed, as expected from its design, of two p-ethylcalix〚4〛arene units 1 in cone conformation fused by two Si atoms adopting a tetrahedral coordination geometry with an average Si–O distance of ca 1.61 Å and O–Si–O angle of ca 109.4° with a distance between the two Si atoms of 3.85 Å. These values are similar to those previously observed for other koilands 〚26–32〛. In the lattice, in addition to the koiland 2, three p-xylene molecules 3 are present: two of them are located within the two cavities (each cavity contains one p-xylene molecule 3 with the shortest carbon to carbon distance of ca 3.45 Å), thus acting as stoppers, whereas the third p-xylene 3 is located between discrete binuclear complexes, thus occupying empty spaces.
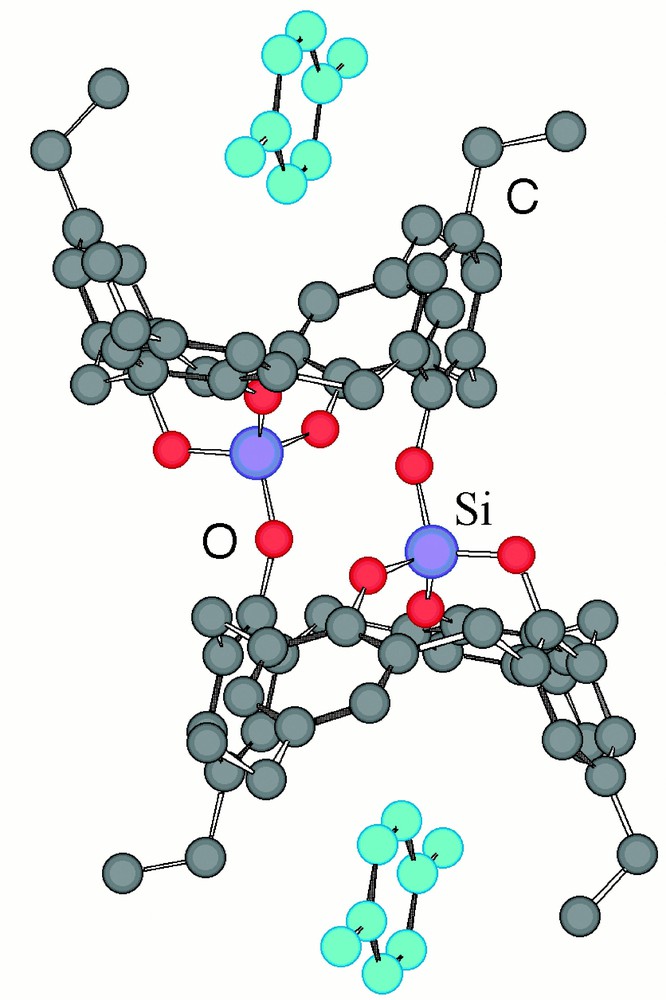
Structure of the exobinuclear complex formed between the koiland 2 and p-xylene 3. For specific distances and angles, see text. H atoms and the third solvent molecule are omitted for clarity.
2.3 Formation of 1-D koilate
Using the koiland 2, the formation of 1-D network based on mainly van der Waals interactions was investigated using 2,4-hexadiyne 4, a rod-type molecule, as symmetrical connector. The latter has been previously used to generate koilates with two other koilands based on p-tert-butylcalix〚4〛arene 〚27〛 and p-i-propylcalix〚4〛arene 〚30〛. Upon slow diffusion of MeOH into a CHCl3 solution of 2 and 4 in large excess (200-fold), suitable colourless single crystals were obtained and studied by X-ray diffraction. In the crystalline phase, the self-complementary koiland 2 forms a linear koilate through self-interconnection of consecutive koilands 1 through inclusion driven mainly by van der Waals interactions (Fig. 4). The assembling core leading by a single translation to the 1-D koilate may be identified as the inclusion of one of the four ethyl groups located at one of the two faces of a koiland 2 by one of the two cavities of a consecutive koiland 2 and vice versa (Fig. 4a). Within the assembling core, a total of 14 van der Waals contacts is present. Among those, the shortest distance of ca 3.45 Å is obtained between the CH3 moiety of the ethyl group acting as connector and the cavity belonging to the next unit, indicating a rather high degree of inclusion. The CHCl3 solvent molecules, although present in the crystal, do not participate in the formation of the self-inclusion network. Surprisingly, although 2,4-hexadiyne 4 is present in the crystal lattice, in marked contrast with previously observed situations based on either p-tert-butylcalix〚4〛arene 〚27〛 or p-i-propylcalix〚4〛arene 〚30〛, for which the compound 4 was acting as a connector; in the present case, it does not play any active role in the formation of the network. However, when looking at the packing of 1-D networks, the 2,4-hexadiyne 4 occupies the empty space between koilates (Fig. 4b).
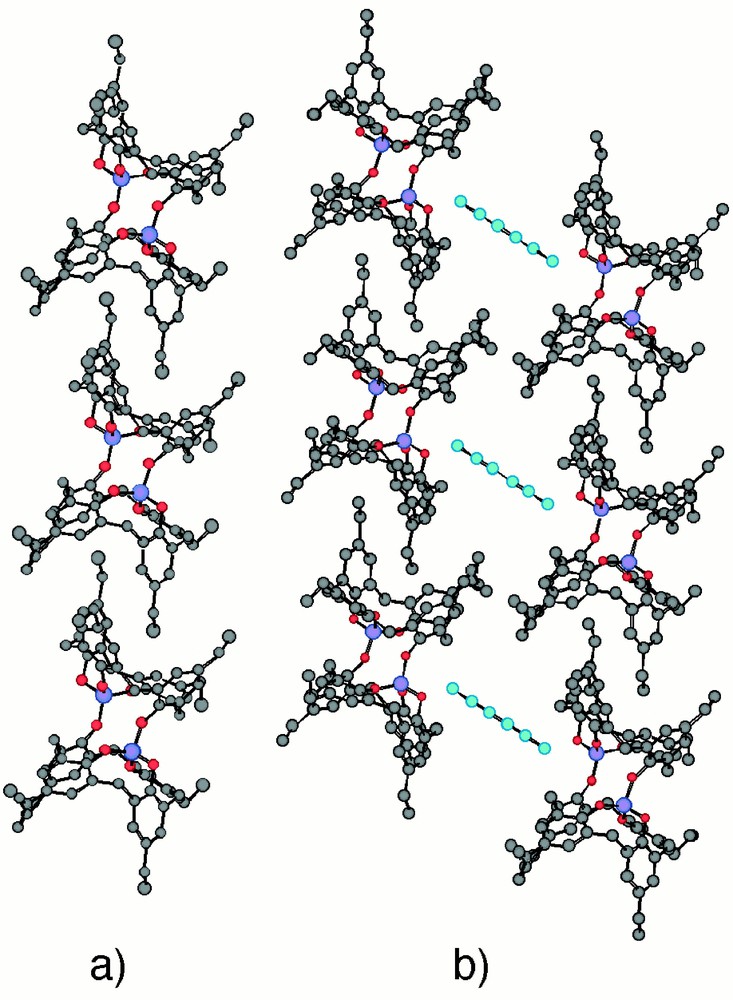
A portion of the X-ray structure of the self-inclusion 1-D network formed by the koilands 2 (a) and the packing of consecutive 1-D inclusion networks generating empty spaces occupied by 2,4-hexadiyne 4 (b). For specific distances and angles, see text. H atoms and CHCl3 molecules are omitted for clarity.
2.4 Formation of 2-D koilate
Rather unpredictably, when the koiland 2 was crystallised from a mixture of benzene 5 and isopropanol, a 2-D self-inclusion koilate was obtained (Fig. 5). The two-dimensional network is formed by double inclusion, by each cavity of the koiland 2, of two ethyl groups belonging to two consecutive koilands 2. The double inclusion of the two ethyl groups leads to 10 van der Waals contacts, with the shortest distance between the terminal CH3 of the ethyl group and the cavity belonging to the next unit of ca 3.56 Å. This rather short distance again indicates a high degree of inclusion. As in the case of the 1-D network mentioned above, the benzene molecules 5 present in the lattice do not participate in the formation of the inclusion network but rather occupy the empty space within the 2-D networks.
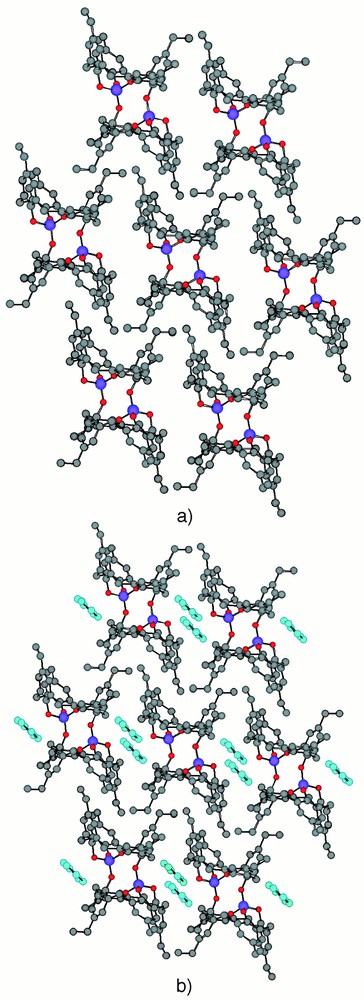
A portion of the X-ray structure of the self-inclusion 2-D network formed by the koilands 2 (a) and the occupation of empty spaces by benzene molecules 5 (b). For specific distances and angles, see text. H atoms and CHCl3 molecules are omitted for clarity.
In conclusion, the symmetrical koiland 2, based on double fusion of two p-ethylcalix〚4〛arenes 1 in cone conformation by two silicon atoms, was prepared and structurally characterised in the crystalline phase by X-ray diffraction on single crystal. The koiland 2 possessing two cavities oriented in a divergent fashion was shown to form, in the solid state, either a discrete exobinuclear inclusion complex in the presence of molecules such as p-xylene acting as stopper, or infinite 1-D or 2-D self-inclusion molecular networks, exclusively based on van der Waals interactions. Further research oriented towards the formation of directional self-inclusion networks using non-centrosymmetric koilands is currently under investigation.
3 Experimental section
3.1 Synthesis of 2
Koiland 2. A solution of p-ethylcalix〚4〛arene 〚33,34〛 1 (150 mg, 0.28 mmol) in dry THF (15 ml) was charged with NaH (30 mg, 1.25 mmol) and the mixture was stirred under Ar for 20 h at 20 °C. SiCl4 (0.3 ml, 0.3 mmol) was added in one portion and the mixture was stirred at 20 °C for another 3 h. After the removal of solvent under reduced pressure, CHCl3 was added to the residue and the solid filtered. The mixture thus obtained was purified by column chromatography (SiO2, solid deposit, hexane/CH2Cl2, 7:3) to afford the pure koiland 2 as a white solid in 30% yield (30 mg). M.p. >300 °C. 1H-NMR (CDCl3, 300 MHz, 25 °C): δ 1.07 (t, CH2–CH3, 6H, 7.5 Hz), 1.13 (t, C H2–CH3, 6H, 7.5 Hz), 1.22 (t, C H2–CH3, 12H, 7.5 Hz), 2.46 (m, CH2–CH3, 16H), 3.30 (d, CH2, 4H, 13.4 Hz), 3.37 (d, CH2, 4H, 13.8 Hz), 4.47 (d, CH2, 4H, 13.7 Hz), 4.56 (d, CH2, 4H, 13.2 Hz), 6.75 (s, H arom., 4H), 6.88 (s, H arom., 4H), 6.89–6.92 (AB, H arom., 8H, 2 Hz); 13C-NMR (CDCl3, 50 MHz, 25 °C): δ 15.2, 15.7 (CH3, ethyl), 27.8, 27.9, 28.1 (CH2, ethyl), 33.4, 34.2 (CH2), 127.6, 127.7, 128.7, 129.0 (CH arom.), 129.3, 130.1, 130.2, 132.7, 137.7, 137.9, 139.6, 145.1, 146.2, 148.5 (C–O, C arom.). 29Si-NMR (CDCl3, 99.36 MHz, 25 °C): δ – 112.66 ppm. Anal. calcd for C72H72O8Si2: C, 77.11; H, 6.47; Si, 5.01. Found for C72H72O8Si2·1.5 H2O: C, 76.92; H, 6.72; Si, 5.09.
3.2 Crystallisation
2-p-xylene. In a crystallisation tube (12 cm long, 0.4 cm diameter), iPrOH (3 ml) was slowly diffused at room temperature into a solution of 2 (1.5 mg) in p-xylene (0.5 ml) to afford colourless crystals.
2-2,4-hexadiyne. In a crystallisation tube (12 cm long, 0.4 cm diameter), MeOH (3 ml) was slowly diffused at room temperature into a solution of 2 (1.5 mg) and 2,4-hexadiyne (21 mg; 200 equiv) in CHCl3 (0.5 ml) to afford colourless crystals.
2-benzene. In a crystallisation tube (12 cm long, 0.4 cm diameter), iPrOH (3 ml) was slowly diffused at room temperature into a solution of 2 (2 mg) in benzene (0.5 ml) to afford colourless crystals.
3.3 X-ray structure analysis
Data were collected on a Nonius Kappa CCD diffractometer using Mo Kα graphite-monochromated radiation. The structural determination was achieved using the Nonius OpenMolenN package 〚35〛.
Crystal data for (2-2,4-hexadiyne): (colourless, 173 K), 2 (C72H72O8Si2)·C6H6·2 CHCl3, M = 2555.93 g mol–1, monoclinic, space group P21/n, a = 13.772(8) Å, b = 29.887(1) Å, c = 17.101(6) Å, β = 110.458(5)°, V = 6594(1) Å3, Z = 2, μ(Mo Kα) = 0.233 mm–1, 6662 data with I > 3 σ(I), R = 0.057, Rw = 0.067 (CCDC 181811).
Crystal data for (2-p-xylene): (colourless, 294 K); C72H72O8Si2·3 C8H10, M = 1440.05 g mol–1, monoclinic, space group C2/c, a = 27.047(1) Å, b = 20.793(1) Å, c = 19.3760(9) Å, β = 132.845(5)°, V = 7989(1) Å3, Z = 4, μ(Mo Kα) = 0.103 mm–1, 4377 data with I > 3 σ(I), R = 0.051, Rw = 0.070 (CCDC 181812).
Crystal data for (2-benzene): (colourless, 173 K); C72H72O8Si2·2 C6H6, M = 1277.77 g mol–1, monoclinic, space group p21/a, a = 10.2577(2) Å, b = 26.3729(9) Å, c = 13.1360(4) Å, β = 105.167(5)°, V = 3429.8(4) Å3, Z = 2, μ(Mo Kα) = 0.111 mm–1, 3974 data with I > 3 σ(I), R = 0.063, Rw = 0.091 (CCDC 181813).
Supplementary material
The supplementary material has been sent in electronic format to the Cambridge Crystallographic data Centre, 12 Union Road, Cambridge CB2 1EZ, UK, as cif files Nos CCDC 181811–13, and can be obtained by contacting the CCDC.
Acknowledgements
We would like to acknowledge the ‘Université Louis-Pasteur’ (Strasbourg, France) and the French ‘Ministère de la Recherche et de la Technologie’ for financial support.