1 Introduction
Phosphate glasses are of great technological interest due to specific thermal properties, i.e. low glass transition and dilatometric softening temperatures, and high thermal expansion coefficients, which make them ideal as low-temperature sealing glasses 〚1–5〛. The problem is their low chemical durability, often associated 〚6〛. However, the nitridation ability of these glasses under ammonia atmosphere, demonstrated as soon as 1982 〚7〛, is a suitable way to improve their chemical resistance.
The structure of metaphosphate glasses consists of –PO4–PO4– chains of tetrahedra, interconnected through modifier cations. The middle tetrahedra, named Q2-groups according to Lippma et al.’s notation 〚8〛, have two bridging oxygens (BO), bonded to neighbouring phosphorus atoms, and two non-bridging oxygens (NBO).
During nitridation in ammonia, P–N bonds form at the expense of P–O bonds, nitrogen substituting within PO4 tetrahedra for both bridging and non-bridging double-bonded oxygens to form PO3N and PO2N2 tetrahedra, as proved by 31P Nuclear Magnetic Resonance (NMR) 〚9, 10〛. Moreover, X-ray Photoelectron Spectroscopy (XPS) results have shown that nitrogen atoms exist as two-coordinated, –N=(Nd), and three-coordinated, –N<(Nt) species 〚10–12〛. As a result of the nitrogen/oxygen substitution, the cross-linking density and the covalency of the glass network increase, which improves effectively the glass durability.
Two different models have been proposed to explain the nitridation mechanism. Reidmeyer et al. 〚13〛 have assumed that the N/O substitution is a random process. On the opposite, Le Sauze et al. 〚9〛 have described the nitridation of the Li0.5Na0.5PO3 glass by oxynitride microdomains growing progressively at the expense of oxide ones, where PO2N2 tetrahedra appear by substituting preferentially bridging oxygens shared by a PO4 tetrahedron and a PO3N tetrahedron. In both cases, it is concluded that non-bridging oxygens coordinating modifier cations are not substituted.
The higher field strength of divalent compared to monovalent modifier cations results generally in higher values of melt viscosity, which leads to a limited nitridation, for example when BaO is introduced in the starting glass formulation. PbO is an exception as its introduction provides a low melt viscosity and thus enables incorporation of high nitrogen contents, as shown by Pascual et al. 〚14, 15〛. Moreover, the presence of Pb2+ cations improves the glass chemical durability while maintaining low softening points 〚16〛, thus making lead-containing glasses appropriate materials for soldering applications.
This paper deals with the thermal nitridation in flowing ammonia of alkali and alkali–lead metaphosphate glasses. In addition to a comparison between the nitridation kinetics, 31P Magic Angle Spinning (MAS) NMR and Double Quantum (DQ)-MAS NMR, and XPS results have been used to compare the structural evolution resulting from progressive nitrogen incorporation.
2 Experimental
The oxide-base glass compositions 50 Na2O·50 P2O5 (‘NaPO’), 25 Li2O·25 Na2O·50 P2O5 (LiNaPO) and 12.5 Li2O·12.5 Na2O·25 PbO·50 P2O5 (‘LiNaPbPO’), in mol%, were prepared by melting, according to a previously described procedure 〚17, 18〛. Phosphorus oxynitride glass samples, ‘NaPON’, ‘LiNaPON’ and ‘LiNaPbPON’, were prepared by thermal treatment of the base glasses in flowing anhydrous ammonia, at temperatures ranging from 600 to 750 °C for times up to 96 h, as previously described 〚17, 18〛.
Nitrogen analyses were carried out in a nitrogen/oxygen LECO TC-436 analyser by the inert gas fusion method, with a maximum deviation in the N/P atomic ratio of ±0.02. The nitrogen content was confirmed by direct chemical analysis as NH3, according to the Grekov method 〚19〛.
31P MAS NMR spectra were recorded on a Bruker ASX 100 spectrometer operating at 40.48 MHz (2.34 T). The pulse length was 3.0 μs (π/2) and 120 s delay time was used. The spinning rate was 15 kHz. The 31P spectra were fitted to Gaussian functions, in accordance with the chemical shift distribution of the amorphous state. The precision of the relative component determination was ±0.5%.
31P Double Quantum MAS NMR measurements were performed at 2.34 T, using a previously described procedure 〚20, 21〛. A 4 mm MAS probe was used at a MAS frequency of 12.5 kHz. The BaBa (back to back) sequence was applied, using TPPI (time proportional phase increment) 〚20–22〛. A 3-μs pulse was used (π/2), and a 5 s recycling delay. Sixty-four t1 increments of 80 μs were used, with 32 accumulations per slice.
XPS measurements were performed on a CAMECA RIBER SIA 200 electron spectrometer for multi-technique surface analysis system. This system is equipped with a MAC 2 CAMECA RIBER double stage cylindrical mirror electron-energy analyser. The photon source was a CAMECA SCX 700 dual anode X-ray source. A non-monochromatized Al Kα X-ray source (hν = 1486.6 eV) was used as the excitation source in all cases. A spectrometer energy calibration was made by using the Au 4f7/2 and Cu 2p3/2 photoelectron lines. Due to surface electrostatic charges detected for some samples and resulting in variable retarding effects, all the energy scales corresponding to the XPS spectra reported in this paper were normalized from the energy position of the C1s photoelectron line of atmospheric carbon (CH2)n: 285 eV. The photoemission measurements were carried out on a series of samples before and after removing the surface carbon contamination by ion sputtering. The sputtering was performed for 10 min in the UHV (ultra high vacuum) analysis chamber with a 0.6 keV Ar+ ion beam (30 mA cm–2). The elemental composition of the samples was evaluated using the integrated areas of the core-level peaks O1s, C1s, P2p, Pb4f, Na1s and N1s. The Li atomic concentration could not be measured due to the very low value of the ionisation cross-section for this element.
3 Results
3.1 Nitridation kinetics
Fig. 1 shows the progressive nitrogen enrichment of NaPON, LiNaPON and LiNaPbPON glasses as a function of nitridation time at T = 700 °C. For the three different base glasses studied, the nitrogen content reaches an asymptotic limit, which is different for each composition. The time after which this limit is reached, i.e. the nitridation rate, depends also on the starting glass composition.

Nitridation kinetics at 700 °C of NaPON, LiNaPON and LiNaPbPON glasses. The lines are only a guide for the eyes.
3.2 31P MAS NMR
The 31P MAS NMR spectra of the NaPO3, Li0.5Na0.5PO3 and Li0.25Na0.25Pb0.25PO3 base glasses contain a single peak at –20.7, –21.4 and –23 ppm, respectively. According to Sato et al. 〚23〛, this resonance corresponds to Q2-type sites. From the previously reported chemical shifts, Q2(Na): –19 ppm 〚23〛, Q2(Li): –23 ppm 〚9〛 and Q2(Pb): –24.5 ppm 〚24〛, and considering an average distribution of the cations around the Q2 sites, we calculate Q2 (Li, Na) and Q2 (Li, Na, Pb) values of –21 ppm and –22.8 ppm for the Li0.5Na0.5PO3 and Li0.25Na0.25Pb0.25PO3 glass compositions, respectively, in agreement with the experimental results.
As N/P increases, two new resonances can be observed in the 31P MAS NMR spectra of the nitrided glasses in addition to the Q2-type resonance mentioned above, which are assigned to PO3N (∼–10 ppm ) and PO2N2 (∼0 ppm ) tetrahedra 〚9, 10, 24〛. As an example, Fig. 2 shows the 31P NMR spectra of LiNaPbPON glasses.

31P MAS NMR spectra of Li0.25Na0.25Pb0.25PO3–3x/2Nx glasses (0 ≤ x ≤ 0.69).
For the three studied series, PO3N and PO2N2 tetrahedra appear at the expense of PO4 tetrahedra. Fig. 3 shows the variation of the relative proportions of the three kinds of tetrahedra as a function of the N/P ratio.
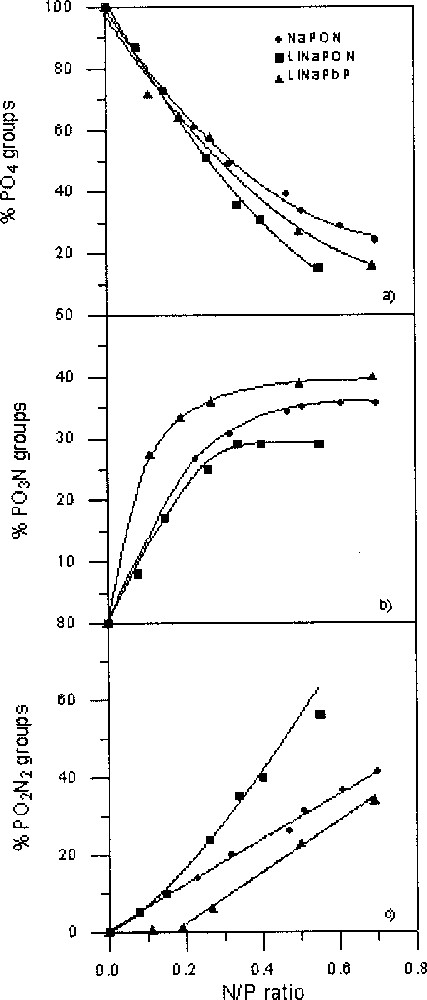
Variation of the percentages of P(O,N)4 units as a function of the N/P ratio in NaPON, LiNaPON and LiNaPbPON glasses: (a) PO4, (b) PO3N and (c) PO2N2 tetrahedra. The lines are only a guide for the eyes.
The PO4 percentage (Fig. 3a) does not decrease at the same rate as the N/P ratio increases. For N/P < ∼0.2, the proportion of remaining PO4 tetrahedra is similar for the three glass series. At higher N/P, it follows the sequence NaPON > LiNaPbPON > LiNaPON.
As shown in Fig. 3b, PO3N tetrahedra appear as soon as nitrogen is incorporated within the glass network, and, in all cases, their proportion versus N/P increases asymptotically up to a maximum value, which depends on the starting glass composition. In the alkali phosphate glasses, the percentages of PO3N are similar for N/P < ∼0.3, then diverge at higher nitrogen contents to reach a maximum around 36% and 29% in NaPON and LiNaPON glasses, respectively. In LiNaPbPON glasses, much higher percentages of PO3N are observed from the beginning of nitridation, with a final value of ∼40%.
The three glass series show also a different evolution of their PO2N2 tetrahedra when the nitrogen content increases (Fig. 3c). In NaPON and LiNaPON glasses, the percentages of PO2N2 only coincide at low nitrogen contents (N/P ∼ 0.15), their increase following a straight line for NaPON with a maximum around 41% for N/P ∼ 0.7, whereas much higher percentages, around 56% for N/P = 0.55, are reached in LiNaPON glasses.
On the other hand, in LiNaPbPON glasses the PO2N2 proportion remains close to zero for N/P < ∼0.2, then increases rapidly up to a maximum of ∼34% for N/P = 0.69. Whatever the considered system, the slope of the corresponding variation curve is never decreasing, even at high nitrogen contents.
3.3 31P DQ-MAS NMR
The 31P DQ MAS-NMR spectrum of the Li0.5Na0.5PO2.17N0.55 nitrided glass sample, which is the LiNaPON nitrogen-richest composition, is presented in Fig. 4a. In addition to an auto-correlation peak A–A, at approximately –40 ppm, which is characteristic of a dipolar connectivity between PO4 tetrahedra, it indicates connectivity between PO3N and PO2N2 tetrahedra (auto- and cross-correlations at –5, –15 and –22 ppm). It reveals also A–B and A–C cross-correlations, i.e. the existence of direct links between pure oxygenated PO4 tetrahedra and partially nitrided ones.
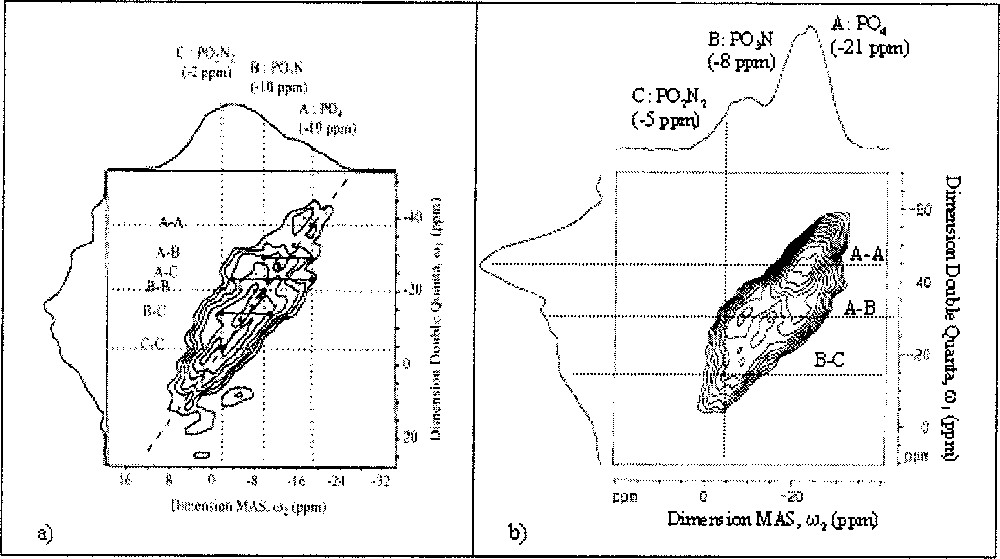
31P DQ–MAS NMR spectra of (a) Li0.5Na0.5PO2.17N0.55 and (b) Li0.25Na0.25Pb0.25PO2.25N0.50 glasses.
The 31P double quantum spectrum of the Li0.25Na0.25Pb0.25PO2.25N0.5 glass (Fig. 4b) shows several differences compared to the LiNaPON glass. It shows a contribution on the diagonal at –21 ppm in the MAS dimension, which can be assigned to PO4–PO4 auto-correlation. The two off-diagonal resonances at –22 and –8 ppm can be attributed to cross-correlation peaks due to connectivity between PO4 and PO3N tetrahedra. Two other off-diagonal resonances are also detected at –8 and –5 ppm and attributed to PO3N–PO2N2 cross-correlations. At high nitrogen content, N/P = 0.69, the 31P DQ-MAS NMR spectrum (Fig. 5) shows that some PO4–PO4 connections still remain along with off-diagonal peaks at –7.5 and –3 ppm, corresponding to PO3N–PO2N2 connections. No auto-correlations PO3N–PO3N or PO2N2–PO2N2 are observed in the LiNaPbPON glasses, even at the highest N/P ratios. In addition, a weak resonance is also detected on the isotropic projection, with a chemical shift of +4 ppm in the MAS dimension, which is attributed to the presence of Q1 sites of pyrophosphate-type 〚25〛.

31P DQ–MAS NMR spectrum of the Li0.25Na0.25Pb0.25PO1.97N0.69 glass.
3.4 N1s XPS
Fig. 6a,b shows the N1s XPS spectra of two representative oxynitride glass compositions, Li0.25Na0.25Pb0.25PO1.97N0.69 (Fig. 6a) and Li0.5Na0.5PO2.17N0.55 (Fig. 6b), which can be decomposed into two Gaussians located at ∼ 397.7 and ∼ 399.4 eV. They have been previously assigned to the two different bonding states of nitrogen, i.e., respectively, –N=(Nd) nitrogen atoms, bonded to two phosphorus atoms, and –N<(Nt) nitrogen atoms, bonded to three phosphorus atoms 〚11, 12, 26, 27〛.

N1s XPS spectra of (a) Li0.25Na0.25Pb0.25PO1.97N0.69 and (b) Li0.5Na0.5PO2.17N0.55 glass compositions.
The variation of the Nd/P and Nt/P atomic ratios versus increasing N/P (as obtained by XPS) is shown in Fig. 7a and 7b for the three glass series. Both types of nitrogen are always present from the beginning of nitridation, and in all cases the variation of Nd/P or Nt/P follows the same trend: the Nt atoms are first predominant before to become in a minority, because the slope of their variation curve decreases progressively with increasing N/P, whereas that of Nd regularly increases.
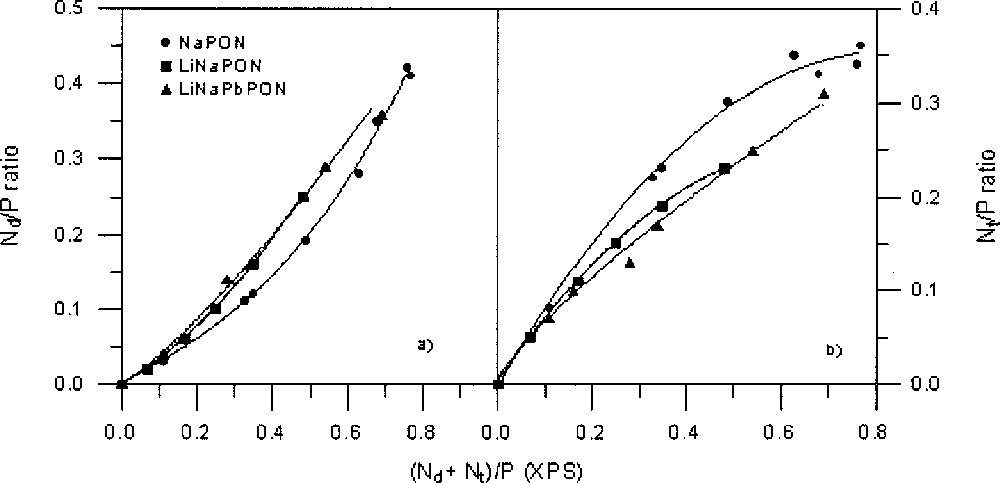
(a) Nd/P ratios and (b) Nt/P ratios, versus total nitrogen content, taken from XPS data, for the three NaPON, LiNaPON and LiNaPbPON glass systems. The lines are only a guide for the eyes.
As can be seen in Fig. 8, the variation of the Nt/Nd ratio depends on the nature of the glass. Whereas a practically linear decrease is observed for the NaPON glasses, the mixed-alkali glasses, LiNaPON and LiNaPbPON give a similar variation, with in both cases a fast decrease during the first nitridation stage.
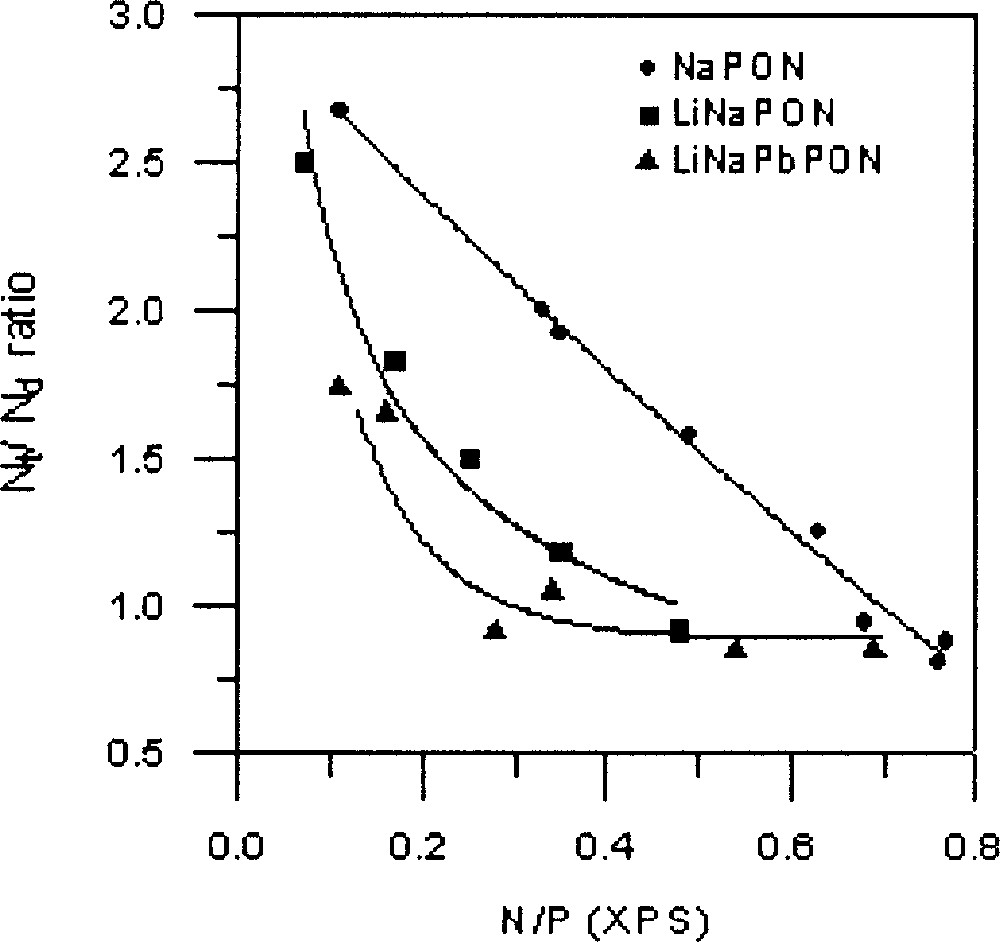
Nt/Nd ratio versus total nitrogen content, taken from XPS data, for the three NaPON, LiNaPON and LiNaPbPON glass systems. The lines are only a guide for the eyes.
4 Discussion
Fig. 1, which represents the nitridation kinetics, clearly shows a dependence on the base glass composition. On the one hand, the time after which the maximum nitrogen content is reached is shorter for LiNaPON and LiNaPbPON glasses, which have lower glass transition temperatures, and therefore lower melt viscosities, than NaPON glasses 〚18, 23〛, which is in accordance with a diffusion-controlled nitridation mechanism. On the other hand, the value of the maximum is clearly a function of the glass composition. In particular, although the nitridation rate is higher in the less viscous LiNaPON glasses than in NaPON glasses, their maximum nitrogen content is lower.
For the three systems, the proportion of PO3N tetrahedra as a function of the nitrogen content (Fig. 3b) reaches an asymptotic maximum value and, since that of PO4 tetrahedra decreases regularly along the whole oxynitride domain (Fig. 3a), it looks like that, from a certain N/P value, PO2N2 tetrahedra form directly at the expense of PO4. The interconnections between the different P(O,N)4 tetrahedra, observed by DQ-MAS NMR, imply existence of both pure oxide and oxynitride microdomains within the glass network. So, the limit in the PO3N percentage can be explained by a nitrogen/oxygen substitution affecting preferentially bridging oxygen atoms shared by a PO4 tetrahedron and a PO3N tetrahedron at the boundaries between microdomains. It results in a simultaneous formation of both a PO3N and a PO2N2 units. In other words, formation of one PO2N2 tetrahedron at the expense of a PO3N is accompanied by formation of another one PO3N at the expense of a PO4, and, consequently, the number of PO3N tetrahedra remains constant. According to this scheme, the N/O substitution would not be a random process during the intermediate and final nitridation stages. However, a purely random substitution cannot be excluded during the first stage.
This nitridation process turns different in LiNaPbPON glasses, which is attributable, of course, to lead atoms. The fast increase in the proportion of PO3N tetrahedra with increasing N/P, together with an absence of PO2N2 before the maximum PO3N percentage is reached, indicates their preferential formation at the beginning. In addition, there are neither PO3N–PO3N nor PO2N2–PO2N2 connections in the corresponding DQ–MAS NMR spectra. The scheme that we propose in this case 〚24〛 assumes that, at first, PO4 tetrahedra located in the vicinity of lead atoms are preferentially nitrided and rapidly transformed into PO3N. Only once all of these PO4 are converted into PO3N units, the other PO4 tetrahedra as well as the PO3N are substituted to form PO3N and PO2N2 units, respectively. The high PbO concentration of the base glass along with the high coordination number of Pb2+ ions would explain the fast increase in PO3N tetrahedra, as well as their higher percentage at the highest N/P ratios than in the NaPON and LiNaPON glasses.
XPS results show that the Nt species are systematically predominant at the beginning of nitridation. However, the Nt/Nd ratio decreases as a function of nitrogen content, showing a different behaviour depending on glass composition (Fig. 8). The decrease is faster for LiNaPbPON glasses. As it has been already proved 〚17〛, the Nt/Nd ratio does not depend on the melt viscosity, but it should be better correlated with structural aspects induced by the nature of modifier cations. At the beginning of nitridation, the formation of Nt nitrogen atoms, assumed to only affect bridging oxygen atoms (BO), proceeds more rapidly, probably because it is easy to replace BO atoms after the cut of P–O–P bonds within the metaphosphate chains. On the opposite, formation of Nd nitrogen atoms requires both bridging and non-bridging (NBO) oxygen atoms 〚12〛. The progressive decrease observed in the formation rate of Nt atoms is explainable by steric effects resulting from the higher cross-linking they induce compared to Nd atoms. The faster decrease of Nt/Nd in LiNaPON and LiNaPbPON than in NaPON glasses could indicate that the greater structural complexity of a multi-cation glass network, associated to the high coordination number of large modifier cations such as Pb2+, is favourable to the formation of Nd species. Further work is now in progress to clarify the structural role of lead by introducing different PbO contents in the Li–Na–Pb–P–O–N system.
On the other hand, the presence in the lead-containing glasses of PO3N tetrahedra as the only nitrided structural units up to N/P ∼ 0.2 confirms that these PO3N tetrahedra form from the two kinds of nitrogen, Nd and Nt, since both species coexist from the very beginning of nitridation. An ideal situation for a complete understanding of the nitridation mechanism would be, of course, to closely correlate the NMR and the XPS data, for example to be able to differentiate a PO3N tetrahedron containing a Nd atom from a PO3N containing a Nt atom, and to distinguish also between the three different possible PO2N2 tetrahedra.
5 Conclusion
In this study, a comparison was made between three different series of oxynitride phosphate glasses, NaPON, LiNaPON and LiNaPbPON, concerning their ability to nitridation and their structure, investigated by NMR and XPS. The results show that, if all the glasses are composed of PO4, PO3N and PO2N2 coexisting tetrahedral units, with both two- and three-coordinated nitrogen atoms, they can present significant differences which originate either from different melt viscosities or from structural aspects related to the nature of the modifier cations.
The nitridation mechanism proposed for LiNaPON glasses, assumed as an initial random N/O substitution followed by a progressive growing of nitrided microdomains from oxide ones, changes with introduction of lead. In this case, it is thought that the nitridation is not random since its beginning. The Pb2+ ions induce nitrogen/oxygen substitution in all the PO4 tetrahedra surrounding lead atoms, thus giving rise only to PO3N tetrahedra before any formation of PO2N2.
The other relevant difference between these systems is the faster decrease of the Nd/Nt ratio in LiNaPON and LiNaPbPON than in NaPON glasses. This could indicate that the greater is the structural complexity of the glass network and the steric hindrance introduced by large modifier cations such as Pb2+, the easier is the formation of Nd nitrogen atoms.
Acknowledgements
This work was financed by MCYT (Spanish Ministry of Science and Technology) through the project MAT 2000-0952-C02-01, and the Integrated Actions CSIC-CNRS 2000FR0009 and HF-2001-124. The authors wish to specially thank Drs. L. Montagne and R. Berjoan for the NMR and XPS experiments.