1 Introduction
Glycosylated proteins are ubiquitous components of extracellular matrices and cellular surfaces, where their oligosaccharide moieties are involved in extensive recognition phenomena including development, differentiation, morphogenesis, fertilization, immune response, implantation, cell migration, and cancer metastasis [1]. Every cell in every living organism is covered by an abundance of such diverse carbohydrate chains, the glycocalix, whose composition reflects not only cell types but also different cell states. Interestingly, abnormalities in protein glycosylation have often been correlated with specific disease states. This has lead to the development of therapeutic agents designed to interfere with carbohydrate biosynthesis or molecular recognition [2] and carbohydrate-based anticancer vaccines [3].
The complementarity of sugar epitopes and specific protein receptors has also found applications in vectorized drug or probe delivery [4] and in the targeted aggregation of pathogenic species [5].
However, despite the biological relevance of glycopeptide and, more generally, glycoconjugate structures, the preparation of such molecules by chemical synthesis is still a challenging and laborious task.
2 The chemoselective ligation approach
The extensive use of orthogonal protection–deprotection strategies, and the need of stereoselective reactions for glycosidic bond formation, are major problems in the assembly of glycoconjugates [6]. One often adopted methodology calls for the synthesis of glycosylated amino acid building blocks followed by their insertion into peptides [7]. This ‘linear’ approach is labour intensive and does not allow the preparation of different glycoforms through the same synthesis. In addition, the presence of the sugar appendage on the amino acid may not be compatible with existing optimised solid phase peptide synthesis (SPPS) protection and coupling schemes.
An attractive alternative involves the ‘convergent’ approach of the chemoselective ligation between tailor-made unprotected peptides and sugars in aqueous solution. Chemoselective ligation reactions were first described by protein chemists as the coupling of two mutually and uniquely reactive functional groups that are selective for each other and tolerate a diverse array of other functionality, that renders the use of protecting groups unnecessary. These reactions offer advantages similar to enzymatic reactions (activating agents are not required) with the potential of a broader range of substrates for use as coupling partners. For these reasons, chemoselective strategies have recently been extended to sugar–peptide conjugation generating glycopeptide analogues (neoglycopeptides) with non-native sugar-peptide linkages [8]. The chemoselective methodologies developed so far for peptide–sugar conjugation can be classified into two broad categories: the first characterized by the reaction of the carbonyl group of ketones or aldehydes with strong nucleophiles, the second by the addition of sulfidryl groups with a variety of electrophile groups.
3 Carbonyl-based chemoselective glycosylation
The absence of aldehydes and ketones on the side chains of the naturally occurring amino acids inspired a set of chemoselective reactions based on the condensation of the electrophilic anomeric carbon of a reducing sugar (aldehyde group in the cyclic hemiacetal form) with a variety of non-natural nucleophilic groups introduced into the peptide chain.
Oxy-amino groups on amino acid side chain [9, 10] or on the N-terminal end of a non-protected peptide [11] were reacted with the reducing sugar of a non-protected mono or oligosaccharide giving oxime-linked neo-glycopeptides (Fig. 1A). The linear open-chain form of the first attached sugar makes such mimetics quite different from the natural counterpart. The use of an oxy-methylamino group on the N-terminal amino acid [12] or on amino acid side chain [13,14] allowed the preservation of the pyranose cyclic form of the linked sugar (Fig. 1B). This method represents a step forward mimetics that resemble more closely to native β-N-linked glycopeptides, as the conjugation reaction affords stereoselectively the β-anomeric configuration. A tripeptide bearing a hydrazide group was selectively condensed with N-acetylglucosamine thus obtaining an N-linked glycopeptide analogue (Fig. 1C) [9]. In a similar approach, with the reversal of electrophile and nucleophile, a ketone group on an unnatural amino acid side chain was reacted with oligosaccharides bearing aminooxy, hydrazide and semithiocarbazide nucleophiles as appendages at the anomeric position (Fig. 1D–F) [15].

Chemoselective ligation methods based on the reactivity of a ketone or aldehyde with strong nucleophiles.
The non-native sugar-peptide linkages embodied in theses structures might negatively impact biologically relevant structural elements, and therefore might compromise the functional activities. In one functional study, however, an oxime-linked analogue of the antimicrobial peptide drosocin demonstrated activity comparable to that of native glycosylated drosocin [16]. The C-glycosidic mimetic of the Tn epitope [Thr/Ser(O-α-GalNAc)] conjugated to a peptide epitope through an oxime bond in an anticancer vaccine construct (Fig. 1G) was found to be biologically active. In this case, the non-native sugar-peptide linkage did not interfere negatively in the overall process of presentation of the glycopeptide on major histocompatibility molecules [17].
All reactions depicted in Fig. 1 take place with an addition–elimination mechanism and are thermodynamically favoured; in oxyamines, hydrazines and hydrazides, the so-called α-effect causes an increased nucleophile character of the reactive nitrogen, ensuring chemoselectivity in the presence of other proteinaceous nucleophiles as amino, guanidino or imidazole groups.
However, the presence of syn and anti isomers in conjugates 1a, 1c, 1d, 1e, 1f and 1g and the lability to acids of the acyl-hydrazine linkage in derivative 1c still limit the broad utilization of these procedures.
4 Thiol-based chemoselective glycosylation
The second group of chemoselective conjugation reactions includes all reactions between the sulfhydril groups and electrophiles (Fig. 2). The superior nucleophilicity of thiols has been exploited for selective glycosylation. The reaction of a cysteine sulfhydril group with haloacetyl [18], bromoethyl [19], maleimide [20] and disulfide [21, 22] electrophile groups on the anomeric appendage of β-glycosides gave respectively products 2a, 2b, 2c and 2d depicted in Fig. 2.

Chemoselective methods based on the addition of sulphur nucleophiles on electrophile groups.
In a different approach, thioglycosides with β-anomeric configuration were used as nucleophiles in the reaction with peptides containing non-natural aminoacid bearing electrophile groups on side chains. Dehydroalanine residues obtained from oxidative elimination of selenocysteines gave conjugation by Michael addition with unprotected thioglucose [23] (Fig. 2E). An obvious drawback of this method is the lack of diastereoselectivity at the peptidic α-carbon atom that provides two diastereomers. On the other hand, compound 2e is more similar to natural O-linked glycopeptides than other derivatives 2a–d obtained by chemoselective ligation.
1-Thio-α and β-D-glucose and 1-thio-N-acetyl-β-D-glucosamine were reacted with L-serine- and L-threonine-derived cyclic sulfamidates as electrophiles affording S-linked glycopeptides (Fig. 2F) [24]. The cyclic sulfamidate amino acids cannot withstand the basic conditions of Fmoc removal, so these amino acids cannot be incorporated within the interior of a peptide during SPPS and thiosugar conjugation is currently limited to the N terminus.
An alternative strategy to the direct sugar-peptide chemoselective conjugations described so far, has been applied to the synthesis of O-linked glycopeptides, and consists in the chemoselective extension of the oligosaccharide chain in a preformed glycopeptide. Glycopeptides containing the GalNAc(α1-O)Ser/Thr amino acid have been assembled by classic SPPS, then a chemoselective ligation point has been generated on the sugar that has been converted into the corresponding C-6 aldehyde by oxidation with the enzyme galactose oxidase (Fig. 3A). Mono- and disaccharides bearing the oxyamino appendage in anomeric position have finally been selectively condensed to the glycopeptide [25]. Analogues of GalNAc bearing a thiol group on C-3 have been incorporated in glycopeptides and then reacted chemoselectively with N-bromoacetamido sugars (Fig. 3B) [26].
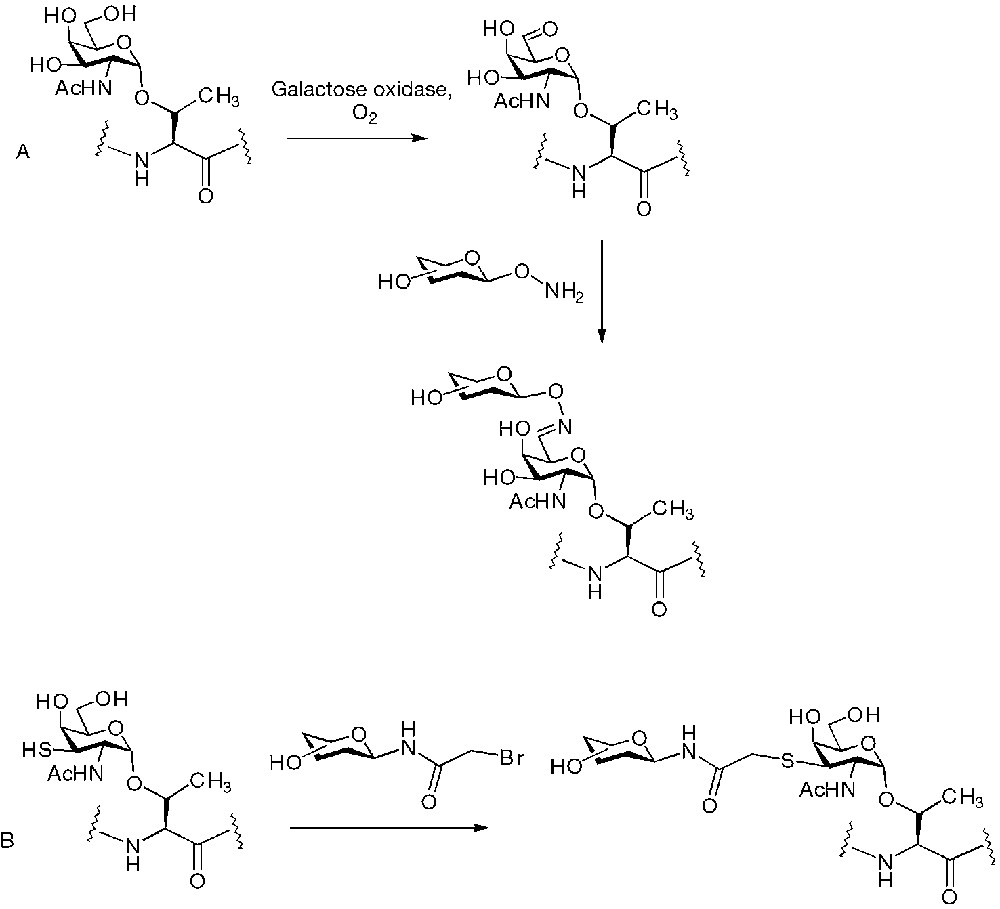
Saccharide chain extension for the synthesis of mimetics of the anti-microbial glycopeptide drosocin.
5 Enzymatic glycosylation
A novel methodology for the preparation of N-linked glycopeptides has been proposed, where the chemoselectivity was achieved by enzymatic reaction [27]. The addition of suitably derivatized oligosaccharides to proteins was obtained using the microbial enzyme glutaminyl-peptide γ-glutamyl transferase (transglutaminase, Tgase). This enzyme cross-links proteins by catalysing an acyl-transfer reaction. The TGase is specific for the glutamine γ-carboxamide group, which acts as the acyl donor. The study showed that the enzyme accepts a wide range of primary amines, thus allowing the construction of a N-neoglycopeptide, the oligosaccharide moiety of which was tethered through an amino-terminated spacer [28]. A very simple and direct method for the functionalisation of the sugar backbone was proposed (Fig. 4); N-acetylglucosamine, maltose, cellobiose, and lactose were derivatized into the corresponding β-N-acetyl allyl glycoside, which were then functionalised by photochemical coupling with cysteamine. Finally the peptide and the sugar were connected by chemoselective enzymatic reaction by transglutaminidase.

Chemoselective glycosylation of peptides mediated by the enzyme TGase.
6 Engineered glycoproteins
Till now, we focused on the synthesis of neoglycopeptides by chemoselective ligation reactions, but recently, new methods for the chemoselective modifications of proteins have been proposed. In fact, site-specific incorporation of probes and natural modifications into proteins can be of great use in biochemical and biophysical studies. Post-translational modifications such as glycosylation are heterogeneous or transient, which complicates the isolation from natural sources of proteins with such modifications, and makes desirable to find other sources. Development of simple methods that allow the incorporation of site-specific synthetic and natural modifications is therefore of great interest for biochemical and biophysical studies.
The use of intein-mediated chemoselective protein ligation strategy was proposed for the synthesis of neoglycoproteins and proteins containing various molecular probes [29]. The method relies on conjugating ‘expressed’ protein bearing a thioester group on the C-terminal end with simple cysteine derivatives [30] following the native ligation methodology developed by Kent and co-workers. Using a variety of synthetic cysteine derivatives, the authors incorporated biotin, fluorescence labels, nucleotides and carbohydrates onto the C-terminus of a 392-residues bacterial protein. In particular, glycosylation of maltose-binding protein (MBP) from E. coli was obtained by expression of the fusion protein MBP-intein, and subsequent cleavage by a suitable glycosylated cysteine derivative (Fig. 5).

Reaction of the fusion protein MBP-intein with glycosylated cysteine affording glycosylation after chemoselective intein displacement.
Bertozzi and co-workers proposed a similar approach, using expressed protein ligation for the synthesis of glycoproteins bearing mucin-like, O-glycosylated, domains [31].
An interesting use of chemoselective ligation was reported for modulating cell surface glycosylation by metabolic interference [2]. This approach, defined also as cell surface engineering, takes advantage of chemoselective ligation reactions designed to modify only one cellular component among all others present in the cell. In order to achieve this result, the two participating functional groups must have finely tuned reactivity, so that interference with coexisting functionalities is avoided. Ideally, the reactive partners would be abiotic, form a stable adduct under physiological conditions, and recognize only each other while ignoring their cellular surroundings. The demands on selectivity imposed by cells preclude the use of most conventional covalent reactions, and thus far only two have proven utility in a biological environment. One chemoselective ligation reaction for engineering cell surface is that between a ketone and an aminooxy or hydrazide group. Bertozzi and co-workers introduced the keto functions onto cells through unnatural sialic acid biosynthesis [32]. Human cells metabolize the unnatural precursor N-levulinoylmannosamine to the corresponding keto-sialic acid residue on cell surface conjugates. Chemically orthogonal to native cell surface components, the ketone can then react selectively with externally delivered amino-oxy or hydrazide reagents to form stable covalent adducts. Application of this reaction includes the chemical construction of new glycosylation patterns on cells [33], new approaches to tumour cell targeting [34], and novel receptors for facilitating viral-mediated gene transfer [35]. The Staudinger reaction has also been used for cell surface engineering: this is the reaction between a phosphine and an azide group to produce an aza-ylide. This unique reactivity was used for the functionalisation of proteins on cell surfaces [36].
7 Chemoselectively assembled synthetic vaccines
Many neo-glycopeptides, synthesized with various chemoselective reactions connecting the sugar and peptide moieties, have been used as carbohydrate-based immunogens in the development of new vaccine strategies.
Extensive work has been reported on the synthesis of clustered glycoside-antigen conjugates by two one-pot, orthogonal chemoselective ligation reactions for selective targeting of the dendritic cell (DCs) mannose receptor [37–40]. Selective targeting of DCs is promising for the improvements in synthetic vaccines strategies. Toward this aim, di-, tetra-, and octavalent glycoside-antigen conjugates have been obtained after two orthogonal hydrazone/thioether ligations, achieved by using thio- derivatives of D-mannose, D-galactose, or D(-)-quinic acid, glyoxylyl (or hydrazino)-N-chloroacetylated lysinyl trees, and N-terminal hydrazino (or glyoxylyl) peptide antigens (Fig. 6).
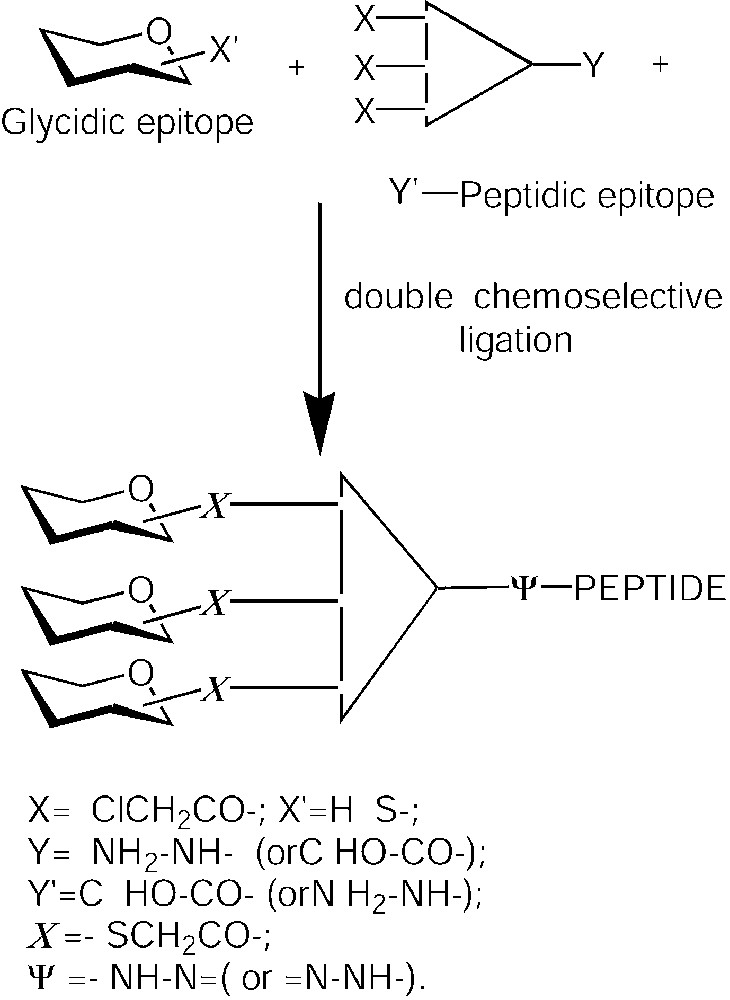
One-pot double chemoselective ligation for the synthesis of vaccines containing clustered sugars.
Anti-tumour vaccines containing an immunogenic peptide linked to one or two copies of the glycidic Tn epitope were synthesized and evaluated in their ability to elicit an immune response both in vitro and in vivo [17,41]. The Tn antigen (GalNAcα-O-Ser/Thr) is over-expressed in various human carcinomas [42] of epithelial origin. In most of these tumours, the Tn antigen, one of the blood group precursors antigens, is found exposed on the external surface membranes, while in all other tissues it is masked and not accessible to the immune system.
Neoglycopeptides 3 and 4 (Fig. 7) were synthesized as potential low molecular weight antitumour vaccines. In these molecules, the peptide OVA(327–339) derived from ovalbumine was covalently linked to one or two sugar B-epitopes. In order to increase chemical and bio-stability, peptide and sugar were connected through a C-glycosidic bond, instead of the natural O-glycosidic linkage. Hence, the fully unprotected α-C-glycosyl analogue of N-acetylgalactosamine 7 [43] was conjugated through an oxime bond to the OVA(327–339) peptide assembled by standard Fmoc solid phase peptide synthesis (Fig. 8) [44]. Neoglycopeptides 3 and 4 were tested in vitro in an antigen presentation assay on dendritic cells (DCs) (Fig. 9). Both neoglycopeptides were presented to T cells and recognized by the T-cell receptor; in addition, it was observed that neoglycopeptide 4, bearing two sugars, was presented to the T-cell receptor with higher efficiency compared to its non-glycosilated analogue. This suggested that the glycidic moieties might either somehow facilitate the internalization of the molecules by interacting with specific receptors, or promote cross-linking of surface receptors, thus causing DC activation. Both phenomena are based on multiple interactions between sugars and receptor and so would be favoured by increasing the number of sugar units clustered on the same molecules.
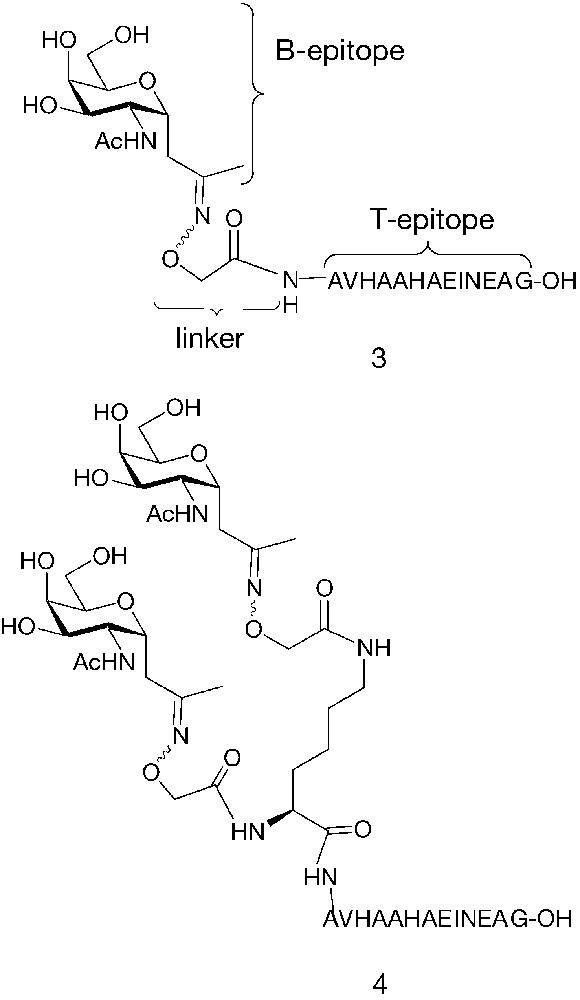
Synthetic vaccines against the Tn epitope-exposing tumours.

Convergent synthetic strategy for the preparation of neoglycopeptides 3 and 4.

Proliferation of CTLL cells dependent of IL-2 production by hybridoma BO 97.10, incubated with both fixed and non-fixed DC: (a) mono-glycosylated neoglycopeptide 3 showed comparable activity than the reference OVA(327–339) peptide; (b) di-glycosylated neoglycopeptide 4 showed a higher response then peptide OVA(327–339) when using non-fixed DC cells.
Indeed, as shown in Fig. 10, the B7.2 molecule (a co-stimulatory molecule expressed by DCs) was up-regulated only in the presence of neoglycopeptide 4. In view of these results, the vaccines were tested in vivo experiments, in order to evaluate the T-cell and the B-cell mediated immune responses (Figs. 11 and 12). Mice C57BL/6 were immunized with non-glycosylated peptide 5, and with neoglycopeptides 3 and 4, mixed with Freund’s adjuvant (CFA). As outlined in Figs. 11A and B, the T cell response (determined ex vivo) after in vivo immunization was highest with the neoglycopeptide bearing two glycidic moieties. In addition, the B cell response was also evaluated (Fig. 12), in order to check if the mice could elicit an immune response against the tumour antigen Tn, by test ELISA. Again, the neoglycopeptide 4, induces the highest antibody production after immunization.

Up-regulation of B7.2 molecule after incubation of DC with compounds 5, 7, 4 and 3. Only neo-glycopeptide 4 induced significant up-regulation of B7.2. The percentage of B7.2 high cells corresponding to the M1 statistical area is reported.

(a) Proliferation of T cell ex vivo, after immunization in vivo with peptide 5, and neoglycopeptides 3 and 4; (b) determination, by ELISPOT, of the production of INFγ of T cell after in vivo immunisation with peptide 5, and neoglycopeptides 3 and 4.
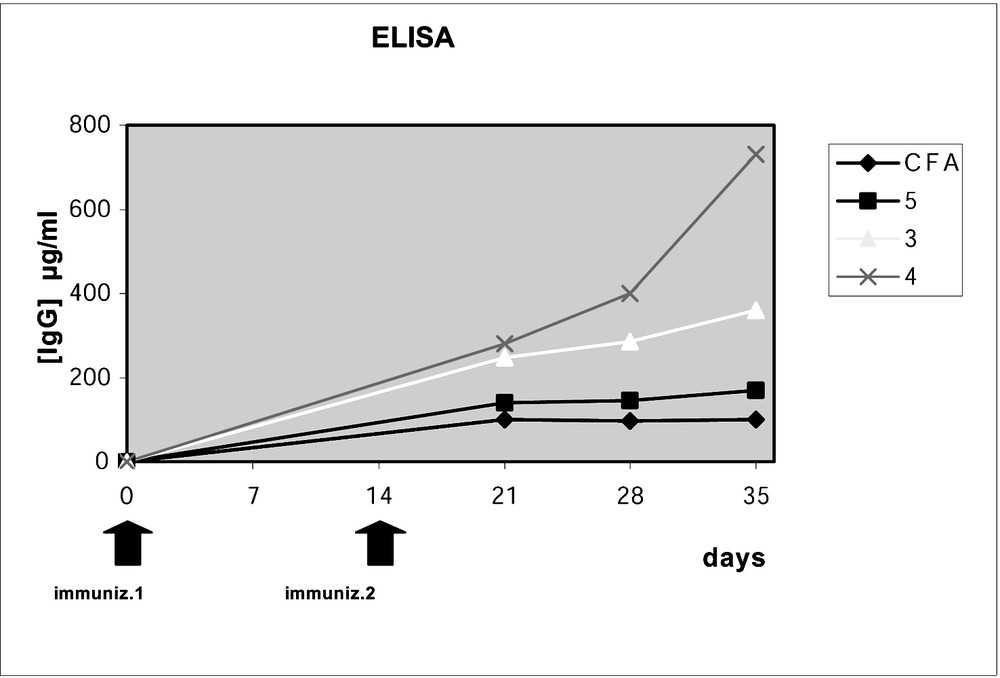
Determination of IgG antibodies by test ELISA of sera after immunisation.
8 Future directions
First developed for the preparation of protein conjugates, the chemoselective ligation chemistry is increasingly applied to the synthesis of other classes of biologically relevant molecules as, for instance, lipopeptides, glycopeptides, glycolipids and oligosaccharides.
The strategy based on the reaction between an aldehyde and an amino-oxy group has been recently applied to the synthesis of oligosaccharide mimetics [45] (Fig. 13). Disaccharide N(OMe) mimetics, isosteres to the native disaccharides, have been prepared in a chemoselective convergent way both in solution and in solid phase by reacting in aqueous media unprotected reducing sugars with monosaccharides bearing an oxyaminomethyl group on C-6.
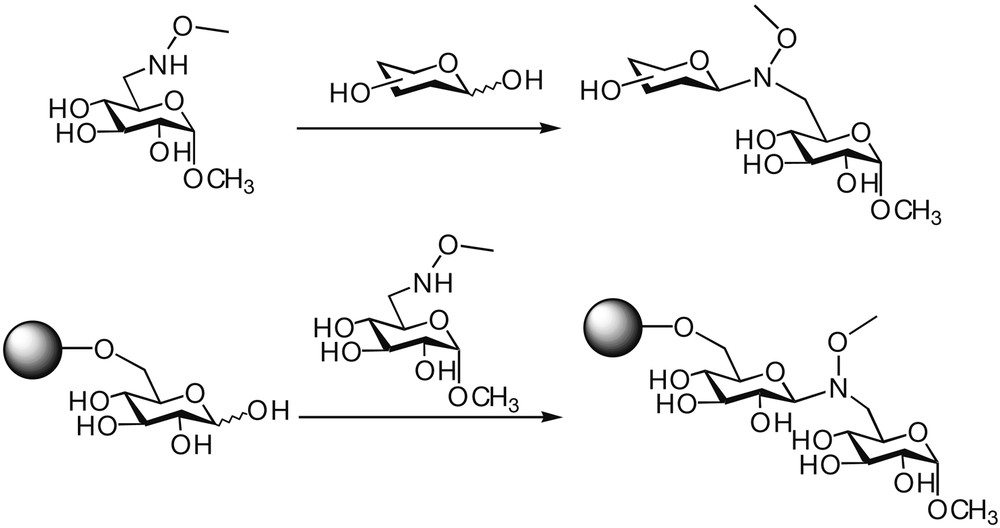
Chemoselective synthesis of N(OMe) disaccharide analogues both in solution and in solid phase.
A major point that should be investigated in the near future is how much the presence of non-native linkers and functional groups in the molecules obtained with chemoselective methodologies is compatible with the molecular recognition phenomena that are responsible for the biological activity and interest of such mimetics.