1 Introduction
The now well-accepted generally globular, or pseudospherical, 3-dimensional architecture of ‘dendritic’ macromolecules [1] leads naturally to a comparison with the idealized concept of traditional micellar organization and related chemistry within their infrastructure. From the perspective of classical, charged amphiphilic aggregation (1; Fig. 1) above the critical micelle concentration (cmc), the original dendritic constructs (2) are structurally similar in that the hydrophilic surfaces connected to more lipophilic interior frameworks are readily attainable. Of course, ‘reversible’ micellar characteristics (i.e., lipophilic exteriors and hydrophilic interiors) are also conceivable and have been demonstrated. Thus, to date, a structurally diverse array of nanoscopic-sized dendrimers as well as their less perfect hyperbranched counterparts has been shown to possess structural parameters comparable to that of supramolecular aggregates known as micelles [2]. Notably, even though in most cases, monomer connectivity is covalent, the iterative nature of dendritic construction imparts an ‘ordering’ to the particular 1→2 or 1→3 branching motifs that create molecular characteristics inaccessible by a collection of simple unordered monomers.
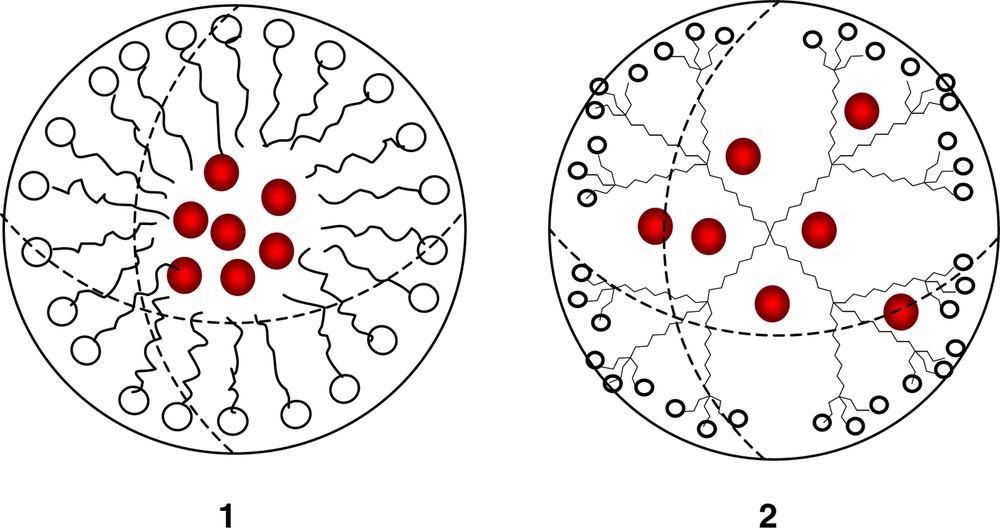
Idealized representations of encapsulated guests in a polymolecular micelle (1) and a unimolecular micelle (2).
However, it is not only from an architectural viewpoint that dendrimers can be contrasted to organized assemblies, but ‘micellar stabilization and organization’ of species in uncharacteristic environments are also suggested. Thus, structural attributes of branched frameworks can be employed for such applications as aqueous solubilization of inherently water insoluble species as well as molecular ordering based on non-covalent interactions, such as H-bonding and ionic associations. The advent and current status of several dendritic micelles are herein chronicled.
2 Supramolecular properties
From the inception [3, 4] of dendrimer chemistry in 1985, and the successful construction of branched, multifunctional architectures, such as polyols 3 and 4 (Fig. 2), the logical application of supramolecular chemistry to this field was obvious, as noted by the original introduction [3] of the term ‘unimolecular micelle’. Such a term denotes the singularity of the molecular structure of the branched host and the unique internal area(s) capable of guest inclusion; thus opening the door for molecular encapsulation and subsequent catalytic properties within these tailored confines. More recently, nanocapsules and nanomachines have been introduced to capitalize on the most novel property of these hyperbranched materials, that is their supra(macro)molecular characteristics – ‘bearing on the organized entities in higher complexity that result from the association of two or more chemical species held together by intermolecular forces’, as defined by Lehn [5]. Thus, molecular encapsulation of guests within the dendritic infrastructure [6–8] permits opportunities for chemical interactions due to their tailorable confines, thus opening the door to nanomolecular machines.
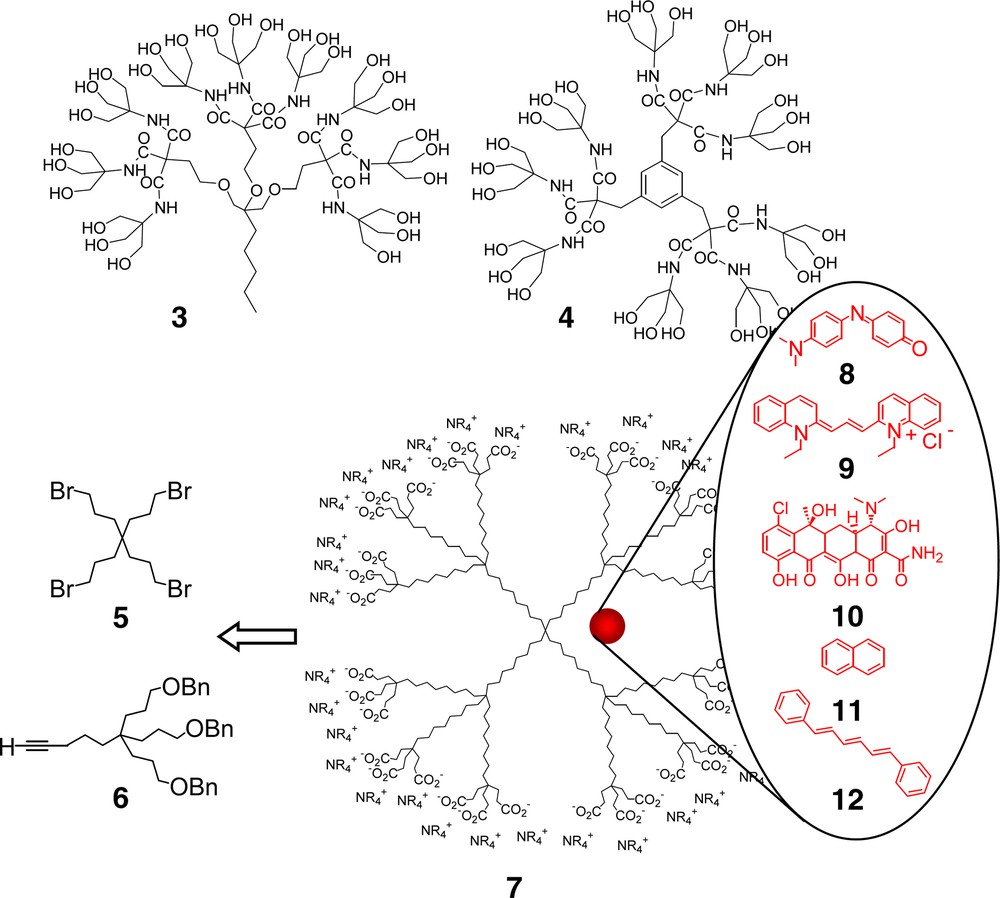
Early ‘Arborol’ constructs (3 and 4) and an all aliphatic hydrocarbon, unimolecular micelle (7).
Although the two-directional, dumb-bell-shaped dendrimers [9–11], based on TRIS construction, were shown to self-assemble into ordered linear arrays, it was the created lipophilic region within the assembly that hosted the fluorescent dye chlortetracycline. It would, however, be several years before the actual proof-of-concept for ‘covalently connected surfactant moieties’ was unequivocally demonstrated [12] in the all hydrocarbon ‘micellane’ dendritic series [13]; this was the first example of an all carbon unimolecular micelle, which incorporated the lipophilic/hydrophilic features of a classical micelle into a single molecule. Pivotal to its construction was access to 1→3 C-branched monomers [14] possessing tetraalkyl-substituted quaternary carbon branching centers via the free-radical-promoted replacement of a tertiary nitro group with an electron deficient alkene (i.e., acrylonitrile or an alkyl acrylate).
Thus, reaction of the tetrabromide core 5 [15] with four equivalents of the requisite terminal alkyne building block 6 via generation of the alkynide anion afforded the 1st generation polyalkyne; concomitant reduction and deprotection yielded the polyol, which was transformed to the corresponding polybromide for iteration. Following reduction and conversion of the periphery to carboxylic acid moieties, aqueous solubility was imparted by treatment with Me4N+OH– to form the poly(ammonium carboxylate) 7.
Molecular encapsulation of traditional probes for micellar properties was demonstrated by the aqueous solubilization, UV, and fluorescence analyses of the guest dyes pinacyanol chloride, (8) phenol blue, (9) chlortetracycline, (10) and naphthalene (11). Fluorescence lifetime decay experiments using 1,6-diphenylhexatriene (12), as the probe, further supported the host–guest relationship; molecular modeling diameters of 48 Å in a fully extended conformation were also substantiated via electron microscopy, which corroborated the single-molecule, non-aggregated state.
During this micellane construction, Tomalia et al. [16] reported the 13C NMR T1 (spin-lattice) relaxation times of 2,4-dichlorophenoxyacetic acid (13) and acetylsalicylic acid (14) in the presence of their PAMAMs [4] (15) and alone in bulk solution (Fig. 3). Since relaxation times were found to be significantly lower with added host the authors suggested either molecular inclusion within the dendrimer or a dendrimer aggregate.
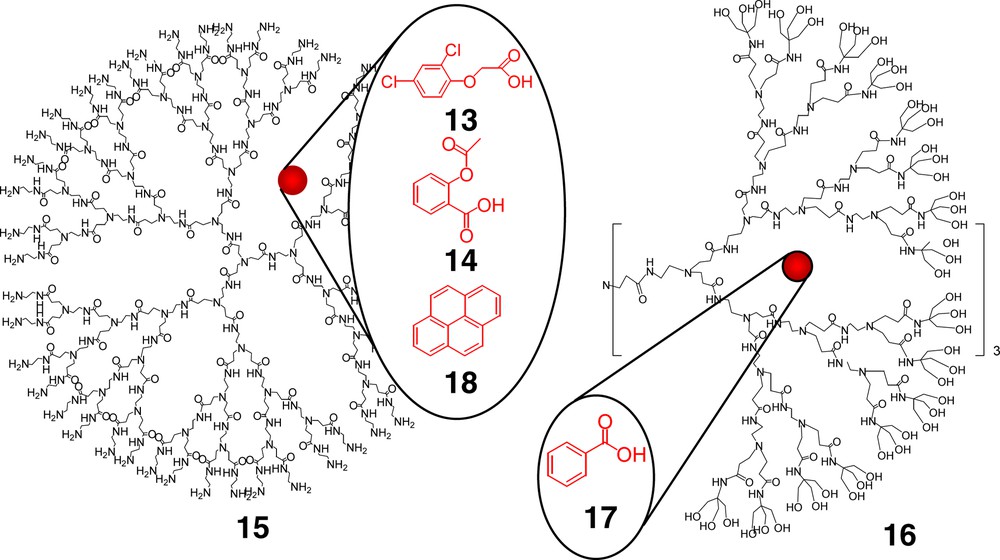
PAMAM dendrimers have been the subject of a variety of micelle-based investigations.
Tris(hydroxymethyl)aminomethane- (TRIS) terminated PAMAMs (16) have recently been created [17] by treatment of the generation PAMAM ester with TRIS via our original procedure [3]. Inclusion complexes with, e.g., benzoic acid (17) significantly increased guest aqueous solubility (300 fold) at neutral pH; while free PAMAM examples denote an acid-base interaction. Turro and coworkers [18, 19] used pyrene (18) in photoluminescent experiments to evaluate internal hydrophobicity and thereby corroborate a predicted morphology change to a globular shape with a more closed and congested surface [16] beyond generation 3.5 for this family. In related studies, PAMAMs constructed starting with lipophilic C12 cores have been exploited as hosts for Nile red dye [20]. Notably, aqueous fluorescence emission was significantly enhanced by the formation of a dendrimer-surfactant assembly upon amphiphile addition. Modification of PAMAM surfaces by treatment with epoxyalkanes [21], such as 1,2-epoxyhexane and 1,2-epoxyoctane, produced ‘nanoscopic container molecules’, which facilitated the solubilization of CuSO4 in toluene vs. a 0.1-M aqueous solution.
Meijer and coworkers [22] have provided a striking example of dendrimer-based, host–guest interaction (Fig. 4) by successfully sterically trapping 3-carboxyproxyl radicals (19), 7,7,8,8-tetracyanoquinodimethane (20; TCNQ), and Rose Bengal (21) within the interior of 5th generation poly(propyleneimine) (PPIs) dendrimers described as a ‘dendritic box’ (22). A filled dendritic box was prepared via reacting the amine surface with an activated ester of a t-BOC-protected amino acid, such as phenylalanine, in the presence of encapsulated guests; notably, complete impermeability of the shell by trapped molecules was observed. Numerous modifications of the surface coatings have appeared instilling a capping property thereby enhancing the host properties, e.g., a peripheral dansyl host surface and the hosted eosin guest [23]. Comparative unimolecular micellar studies of the amino-terminated PAMAMs and PPIs have also appeared demonstrating the importance of internal structure [24–26].
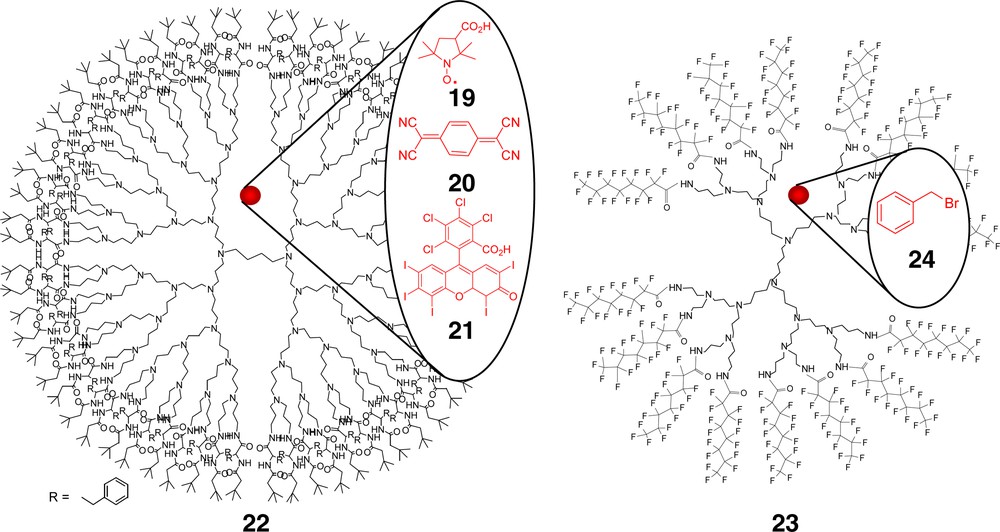
Poly(propylene imine) dendrimers formed the scaffolding for the ‘dendritic box’ (22) and have also been modified to act as reverse micelles.
An excellent example of a ‘reverse unimolecular micelle’ was derived also in the PPI series in which their terminal amines were treated with long chain (C5, 9–15) acid chlorides affording a series of inverted micelles [27]. Ethanol solutions of these dendrimers and Rose Bengal afforded 2nd and 5th generations of host–guest complexes entrapping an average of 1 and 7 (or 8) guests, respectively. Dynamic light scattering experiments supported single particle behavior. Also, coating the surface with palmitoyl chloride and subjection to low pH gave rise to efficient anionic dye extractors [28]; however, higher pH environments (i.e., > 6) afforded less effective extractors. Similar materials have been employed for oxyanion-based extraction of pertechnetate, perrhnetate, AMP, ADP, and ATP [29]. Aliphatically-terminated PAMAM-related dendrimers have appeared and shown to form self-assembled gels based on intermolecular internal H-bonding, thus providing a new methodology to supramolecular nanostructures [30]. As well, host–guest binding constants using pyrene as the probe [31], PPIs quaternized with glycidyltrimethylammonium chloride as pH-sensitive, controlled drug release systems [32], and unimolecular micelles crafted by the preparation of oligo(ethyleneoxy)-terminated PPIs [33] have also been reported.
Van der Broeke and coworkers [34] have created perfluoro-functionalized PPI dendrimers (23) and demonstrated their potential as phase transfer catalysts in supercritical CO2-water mixtures and as anionic species extractants. The dendrimers were accessed via reaction of perfluorinated, linear alkyl acid chlorides with the terminal amines. Extraction of permanganate or dichromate from aqueous to CO2 solution was described as “rather low;” whereas their use as phase transfer catalysts in a SN2 reaction of benzyl chloride to bromide (24) resulted in a high conversion rates.
Dykes et al. [35] reported that peptide-coupled L-lysine amino acid-based dendrimers solubilized proflavin hydrochloride and aurin tricarboxylic acid in CH2Cl2. The magnitude of dye solubilization increased as the degree-of-branching increased, in which at generation 4, incorporation of up to 97% of suspended proflavin·HCl was the best-case scenario. Dye incorporation was enhanced by focal carboxylic acid or amine coordination with a proflavin free amine or an aurin acid moiety, respectively. Control experiments with unbranched hosts, such as acetic acid, showed as expected very little dye uptake further suggesting that “dendritic branching does indeed play a key role in the dye solubilization process”.
Soon after the introduction in 1990 of the convergent route [36] to dendrimer synthesis, hemispherical, aryl ester coated, benzyl ether-based dendrons were reported [37]. Attachment to a divalent core and conversion to the polycarboxylate 25 (Fig. 5) yielded dendrimers, which facilitated a 200 fold increase in pyrene (26) solubility in water compared with that of pure water without the dendrimer. Notably, use of sodium dodecyl sulfate (SDS; above cmc; 9 × 10–3 M) for pyrene encapsulation resulted in only a 100-fold enhancement.
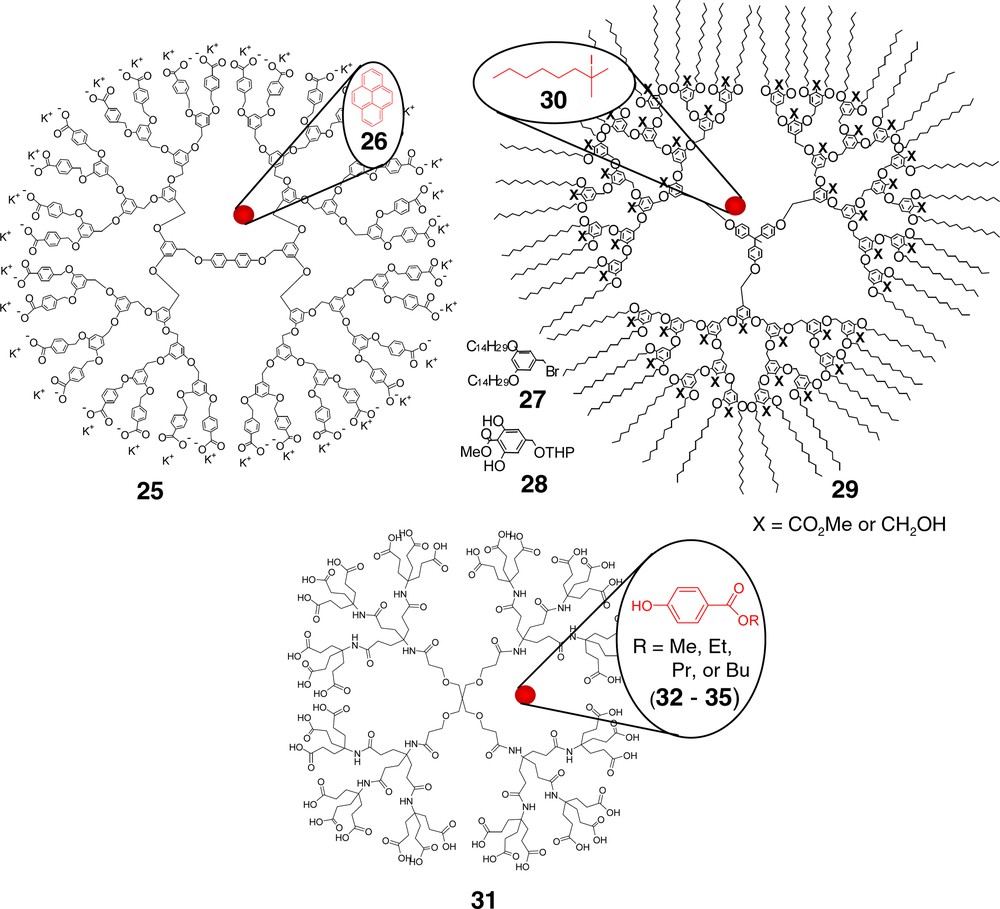
Fréchet’s convergent technology has facilitated the construction of several unimolecular micelles (e.g., 25 and 29), while Newkome’s polyamides have been used as surfactant substitutes in electrokinetic capillary chromatography.
Piotti et al. [38] reported the construction of Fréchet-type reverse unimolecular micelles possessing polar internal aryl methyl esters at each generation and linear C14 alkyl chains at the periphery. Construction began with reaction of 3,5-bis(tetradecylaryl ether) benzyl bromide (27) with the 3,5-dihydroxybenzene (28). Repetition of the standard benzyl alcohol to bromide conversion [36] followed by phenolic substitution of the benzylic halide produced 4th generation wedges that were subsequently attached to a tris(phenolic) core to afford the desired architecture 29. The internal methyl aryl ester groups were also converted to alcohols via LiAlH4 reduction. Micellar potential was demonstrated by use of these dendrimers as catalysts in elimination reactions of tertiary iodoalkanes. The greatest effect was observed for the transformation of 2-iodo-2-methylheptane (30) with a recorded 99% conversion to alkene (dendrimer to substrate ratio, 1:17,600); while the lowest observed conversion was 83%.
Acid-terminated, amide-based dendrimers (i.e., 31) have been shown to be effective surfactant substitutes in the analytical separation technique called ‘micellar electrokinetic capillary chromatography’ (MECC) [39]. The dendrimers were divergently prepared by iterative peptide-type coupling using Behera’s amine [40] based on an a tetrakis(carboxylic acid) [41] core. A series of alkyl parabens (methyl, ethyl, n-propyl, and n-butyl 4-hydroxybenzoate; 32–35) were separated employing 1st–3rd generation polyacids with the higher construct affording the best results. Essentially, the greater the analyte:micellar (i.e., dendrimer) interaction, the longer the analyte retention time in the capillary. Thus, the observed order of elution started with the least lipophilic benzoate and progressed successively to the most lipophilic. Separations were shown to be strongly pH dependent with acidic buffers yielding no results due to dendrimer interaction with the fused-silica capillary surface effectively stopping mobility. The pH dependence for the hydrodynamic radii of this series of dendrimers had been previously demonstrated [42,43]. MECC has also been examined using PAMAM dendrimers as surfactant substitutes [44].
Dendrimer-encapsulated, randomly dispersed metal(0) nanoparticles have been reported [45–56] utilizing either the PAMAM or PPI families [57]. The recent report of substrate-specific hydrogenation of olefins with triethoxybenzamide-terminated PPI dendrimers with encapsulated Pd particles demonstrated the unique catalytic internal environment and the potential as dendritic nanoreactors [58].
3 Internally tailored functionalization
Whereas the majority of dendrimer-based lipophilic-hydrophilic inclusion phenomena discussed thus far results in random guest positioning on a branched framework, ‘ordered’ interactions in supramolecular assemblies are also of interest. The intermediate alkyne precursors to 7 have been treated with B10H14 to afford the 1,2-dicarba-closo-dodecaboranes, thus creating o-carboranes within the dendritic host demonstrating the viability to internal structural modification at specific loci [59]. Expansion of this theme has thus lead Newkome et al. [60] to introduce a single, internal, site-specific, H-bonding-based, molecular recognition site within each dendron component for guest positioning. Incorporation of the binding sites was facilitated by a single-pot, three-component procedure whereby 1,5-glutaryl dichloride was reacted with one equivalent of an aminotris(tert-butyl ester) followed by treatment of an excess of 2,6-diaminopyridine (36a; Fig. 6) to form an extended aminotriester useful in iteration. NMR titration experiments using glutarimide and 3'-azido-3'-deoxythimidine (AZT; 37a) revealed the appropriate downfield shifts of the pyridinyl diamide protons supporting dendrimer-guests complexation at the desired loci. Such examples of internal H-bonding have been limited but their resultant novel chemistry suggests that the supramolecular interactions have far reaching ramifications as noted by recent examples [61–64]. The addition of the paramagnetic 1H NMR probe Co(II) to ligand 36a created a metal-guest complex (37b) at a site specific locus within the dendrimer [65,66]. The piperazine moiety has also been introduced into each dendron and then assembled to afford 36b, then with added Pd(II) or Cu(II), site-specific, host–guest complexation (37c) has afforded the related metallospheres and superclusters [67–70]. Similarly constructed bipyridine-based dendrimers (36c) have also be prepared and shown to form stronger host–guest complexes (37d) [71]. Inclusion of directed heterocycles, e. g., melamine [72,73], within the macromolecular microdomain offers tremendous opportunities to design specific catalytic properties.
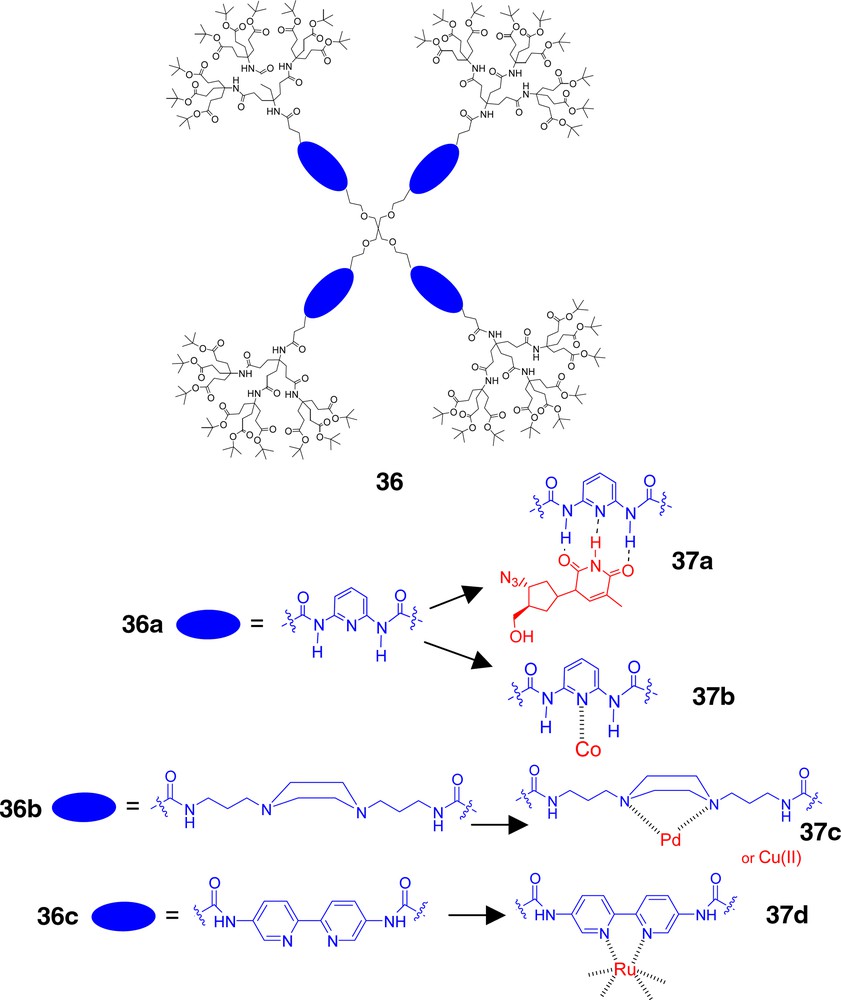
A modular approach to unimolecular micelle construction has facilitated the incorporation of ‘site-specific’ binding loci.
4 Core functionalization
Functionalized cores capable of molecular inclusion and recognition such as the use of calixarenes [74] 38 (Fig. 7) occurred early on in the development of dendrimer chemistry. The first photochromic dendrimers with a 1,3-alternating conformer of a calix [4] arene as a core and azobenzene-containing branches have appeared and were suggested as drug delivery systems [75]. Also, dendrons on β-cyclodextrin core have been demonstrated to self-assemble on a bis-adamantane assembly [76].
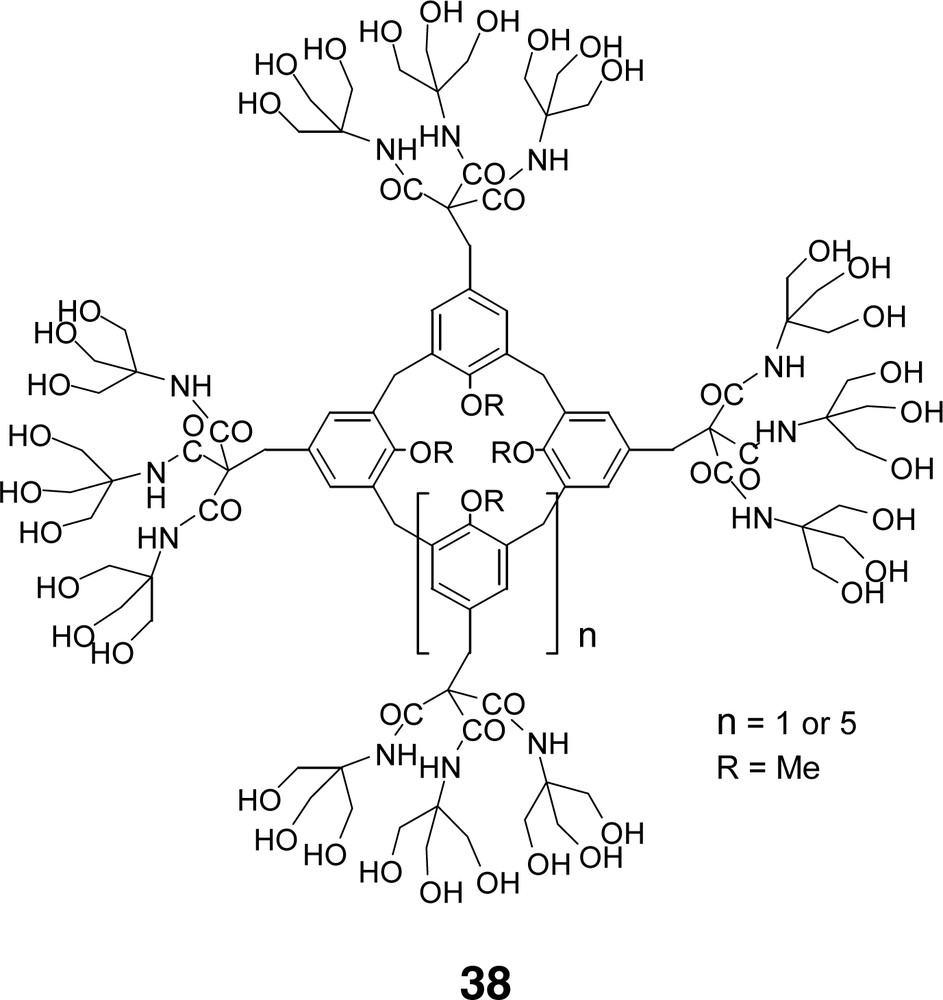
Diverse calixarene chemistry has been incorporated into the micellar regime.
5 Surface tailored functionalization
Surface-functionalization of commercially available dendrimers has opened easy access to understanding the role of terminal moieties in host–guest complexation. González et al. [77] have reported the surface modification of PPIs (e.g., 40) with cobaltocenium groups (Fig. 8) that are easily reduced to Co(0). Reaction of the amine termini with 1-chlorocarbonylcobaltocenium afforded the metal-terminated dendrimers. The lipophilic, charge neutral polycobaltocenes were rendered H2O soluble via addition of β-cyclodextrin (39, β-CD) based on its cylindrical encapsulation of the metallocene moieties 40a. Reversible electrochemical oxidation to the charged state afforded the uncomplexed dendrimer form. Similar ferrocenyl-terminated PPIs are also known [78].
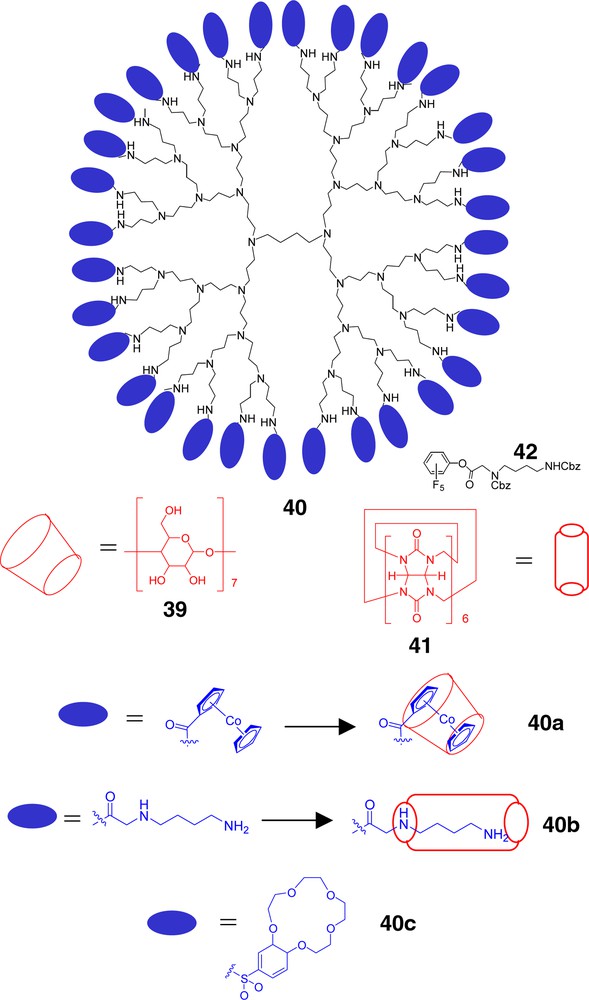
Supramolecular component ordering can easily be achieved by pairing complementary units.
Kim et al. [79] have adapted the periphery of PPIs for a similar, albeit more ionic, coordination with cylindrical cucurbituril (CB; 41) units to give a stable, poly(pseudorotaxane) architecture. The required bis(ammonium)-coated scaffolding was accessed via treatment of the PPI’s amine surface with the perfluorophenyl-activated ester of a bis(protected) diamine-modified acetate 42, followed by deprotection. Dissolution of the polyprotonated framework in H2O, followed by the addition of CB afforded the self-assembled product 40b. Preliminary studies aimed at decomplexation via treatment with base yielded partial separation. T1 (spin-lattice) relaxation experiments with the CB-dendrimer complex showed a sharp increase with higher generations suggesting the outer shell possessed attributes of a ‘solid phase’; such behavior has also been noted by Jansen et al. [22].
Baars et al. [80] reported surface functionalization of PPIs amines with adamantane isocyanate to afforded terminal adamantane surface. Supramolecular surface ordering of carboxylic acid-modified urea guests was achieved based on ionic acid-base association at the outermost dendritic tertiary amine moieties and urea promoted H-bonding. T1 relaxations were found to increase significantly at the complexes surface verifying enhanced rigidity. These adamantly-coated dendrimers were shown to form (1) well-ordered, densely packed self-assembled monolayers with β-cyclodextrin on gold substrates, such interactions suggest a novel route to ‘molecular printboards’ [81] as well as (2) hosts for N-terminal tert-butoxycarbonyl-protected peptides resulting the CHCl3-soluble complexes [82].
Dendrimers possessing internal and terminal crown ether moieties have been known for a decade [83, 84] but the attendant chemistry has only recently started to unfold; the coating of PPIs with benzo[15]crown-5 ethers (40c) has been reported [85] and shown to resemble a unimolecular micelle by preferentially encapsulating the guests within the internal regime.
6 Conclusion
The supramolecular properties of dendritic constructs have been shown to be useful in diverse applications. Host–guest complexation is but the initial stage in the evolutionary process to better understand chemical containment within these complex structures and then to harness these factors in order to develop the specific nanomolecular capsules, machines or devices of the future. Since most of the current studies are based on but a limited number of commercial dendrimers, future endeavors will need to address the availability of more interesting and directed monomers so that the macromolecular researchers will have better tools to harness these very fascinating, inner molecular domains.
Acknowledgements
We gratefully thank the National Science Foundation (DMR 99–01393), Office of Navel Research (N00014–01–1-0856), and the Ohio Board of Regents.