1 Introduction
Since the last century, the tremendous exploitation of the earth petroleum reserves led to a dramatic exhaustion of the carbon sources and researchers estimated to 50 years the remaining time of life of the available fossil carbon. As an alternative to this unavoidable lack of raw materials, our groups focused their investigations towards the use of agroresources, which represent a huge renewable and natural carbon source [1–2]. Among these agroresources, sucrose (SuOH) can be extracted either from sugar cane or sugar beet and our groups particularly studied the non-food industrial applications of this natural polyol [2–4].
The etherification of sucrose by a fatty chain provides a new generation of non-ionic surfactants that could be more biodegradable and biocompatible than those commonly used. In contrast to sucroesters, sucroethers are more chemically resistant in strong alkaline conditions, which considerably extends their potential applications. Several methods of sucroethers preparation have been described either with organic solvent [5–12] or without [13–14].
The direct etherification of unprotected sucrose can be carried out in water with 1,2-epoxydodecane, using tertiary amines as homogeneous catalyst [15,16]. Despite the use of an aqueous solvent, the epoxide hydrolysis remained low by contrast with what was observed with mineral bases such as potassium or sodium hydroxides.
Heterogeneous catalysts offer many advantages, like easy separation of the catalyst from the reaction products [17]. Pure metal oxides [18,19] or impregnated over well-ordered mesoporous silica [20–23], caesium exchanged MCM-41 [25–27] and calcined hydrotalcites [26,27] with different Al/Mg ratios are among the most commonly used. However, most of these solid catalysts require high temperatures, which are not compatible with the sucrose stability.
Basic anion-exchange resins are becoming an area of growing interest, since they have been used as catalysts in organic synthesis [28,29] as phase transfer for the production of cyanides [30] and ethers [31] or in alkylation reactions [32,33]. The easy anion exchange by many basic organic salts such as acetates, alcoholates provides a wide range of resins with a large scale of basicity [34]. Contrary to the oxides previously described, these organic polymers are known to be efficient at low temperature, which makes them attractive catalysts for the sucrose etherification [34].
In this paper, we report the ‘one-pot’ sucroethers synthesis starting from unprotected sucrose and catalysed either by tertiary amines as homogeneous catalysts and basic anion-exchange resins as heterogeneous catalysts (Fig. 1). A better understanding of both homogeneous and heterogeneous catalytic mechanism pathways will be discussed.
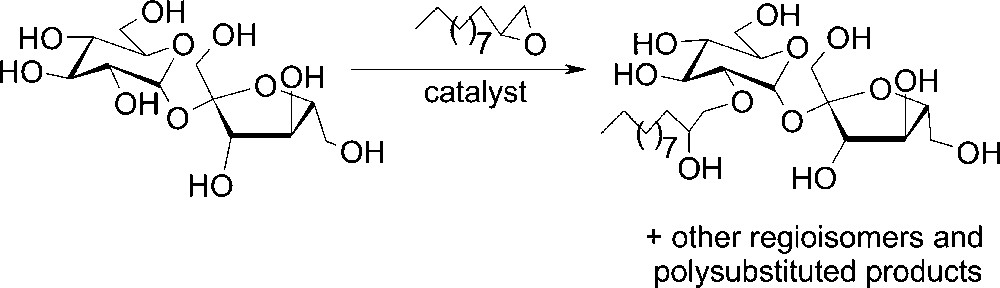
Etherification of sucrose with 1,2-epoxydodecane.
2 Experimental
Both anion-exchange resins A21 and A26 and sucrose were respectively provided by Rohm and Hass and Beghin-Say. 1,2-Epoxydodecane and all different bases were purchased from Sigma-Aldrich and used without further purifications. Anhydrous dimethylsulfoxide was purchased from Fluka and stored over molecular sieves (4 Å).
2.1 Analytical methods
Reaction progress was monitored on a HPLC apparatus equipped with a column Touzart & Matignon Nucleosil C8 250 mm × 4.6 mm, using a mixture methanol/water (78:22) as eluent and a flow of 0.8 ml min–1. This analytical technique allowed quantification of sucrose mono- and diethers, 1,2-dodecanediol and 1,2-epoxydodecane [15–16]. Regiochemistry was also analysed by HPLC (NH2 column). Major functionalisation takes place at 2-OH and 1′-OH. However, this issue will not be discussed in this paper, since no significant difference was observed throughout the study.
Variations of pH were measured starting from a mixture of epoxydodecane (0.3 mmol), amine (0.3 mmol), DMSO (1 g), and water (0.5 g). All pH data were recorded after dilution with 8.5 g of water and compared to pH calculations (Table 1).
pH monitoring after heating amines with epoxydodecane in DMSO/water (1 h, 110 °C)
Base | pKa | pHcalc.a | pHmeas.b | pHmeas.c | ΔpH |
(iPr)2NEt | 11.4 | 11.9 | 11.75 | 11.75 | 0 |
NMM | 7.4 | 9.9 | 9.9 | 10.75 | +0.85 |
DABCO | 8.9 | 10.7 | 10.5 | 12.05 | +1.55 |
2.2 Anion-exchange resins
Anion-exchange resin A21, functionalised with dimethylamino groups, is weakly basic, with a 54–60% water content and a particle size distribution in the range 0.4–1.2 mm. Strongly basic quaternary ammonium type-I anion-exchange resin (OH– form) A26 contains 67–73% of water and a particle size distribution in the range 0.4–1.2 mm. Prior to use, both anion-exchange resins were washed several times with distilled water and dried by extensive washing with 95% ethanol, diethylether and then kept under vacuum at room temperature for 12 h up to constant weight. The anion-exchange capacity for the A21 and A26 resin are respectively 4.70 and 4.40 mmol g–1.
2.3 General procedure for the homogeneous catalytic etherification of sucrose
Sucrose (0.68 g, 2.0 mmol), 1,2-epoxydodecane (0.19 g, 1.0 mmol), base (n equiv vs epoxide, as indicated in tables) and DMSO (3.5 g) were sealed (Teflon-coated septum) in a vial and heated at 110 °C (or other temperatures as stated in tables and figures) under stirring (750 rpm). Reaction progress was monitored by HPLC and yields were calculated with respect to 1,2-epoxydodecane.
2.4 General procedure for the heterogeneous catalytic etherification of sucrose
Under nitrogen atmosphere, 7 g of sucrose (20 mmol) and 0.92 g of epoxydodecane (5 mmol) were added to 10 ml of dry DMSO. The reaction mixture was heated at 110 °C and 0.6 g of dry anion-exchange resin was added. The reaction mixture was stirred at 110 °C and the reaction progress was monitored by HPLC. After complete epoxydodecane consumption, the anion-exchange resin was removed by filtration and thoroughly washed with warm DMSO (80 °C).
2.5 Formation of the ammonium derivatives
Dimethyldodecylamine (6.42 g, 30 mmol), NaHCO3 (3.03 g, 30 mmol) and 45 ml of H2O were heated at 85 °C for 2 h. Epoxydodecane was then added (6.40 ml, 30 mmol), and the mixture was stirred at 95 °C until complete epoxide consumption (monitored by HPLC). The reaction mixture was then dried under vacuum. Analytical data indicated that ammonium was formed with a side production of 19% of dodecanediol and 18% of remaining amine.
1H NMR (MeOD/D2O/acétone-d6 75:25:20 v/v/v) δ (ppm): 0,91 (t, J = 6,7 Hz, 3H, –CH3); 1,20–1,60 (m, 38H, CH2); 3,21 (s, 3H, n-CH3); 3,22 (s, 3H, N-CH3); 3,35 (d, J = 5,6 Hz, 2H, N–CH2–CHOH); 3,40–3,55 (m, 2H, N–CH2–CH2); 4,15 (m, 1 H, CHOH). HRMS (FAB): M+. Theoretical mass: 398,4361. Calculated mass: 398,4368.
Catalytic reactions with sucrose were run according to the following conditions: sucrose (0.54 g, 1.60 mmol), crude ammonium salt (0.50 g, 0.75 mmol) and N,N-dimethyldodecylamine (44 mg, 0.21 mmol) were diluted in water (0.15 g) and heated at 110 °C for 6 h. HPLC analysis and purification by silica gel chromatography [15,16] evidenced the formation of 2% of monoethers.
3 Homogeneous catalysis
In water, very weak bases, such as the N-methylmorpholine (NMM, pKa = 7.4) were surprisingly able to catalyse the reaction, whereas deprotonation of sucrose normally requires stronger bases (pKa of sucrose = 12.6 [35–36]). Moreover, the initial reaction rate was directly related to the base nucleophilicity power and to its affinity for the epoxide phase. Indeed, highly basic hindered amines did not promote the reaction, whereas less hindered bases (methyl substituents or constrained configurations) led to good yields of sucroethers. For instance, the 1,4-diazabicyclo[2.2.2]octane (DABCO) gave the better yields, despite its lower pKa (Table 2). Based on these results, we suggest that the catalytic activity observed could be related to a reversible addition of the tertiary amine on the epoxide, leading to a strongly basic quaternary ammonium salt (Fig. 2), which could act as catalyst and/or emulsifier [15,16].
Catalytic etherification of sucrose in DMSO
Base | Nucleophilicity | pKa | Time (h) | Monoethersa | Diethersa | Diola |
(iPr)2NEt | none | 11,4 | 24 | 0 | 0 | 8 |
N(Pr)3 | none | 11 | 10 | 0 | 6 | |
NMMb | low | 7,4 | 20 | 7 | 0 | 25 |
Me2N(CH2)2NMe2 | moderate | 10,5 | 18 | 39 | 8 | 17 |
DABCO | high | 8,9 | 10 | 51 | 15 | 10 |
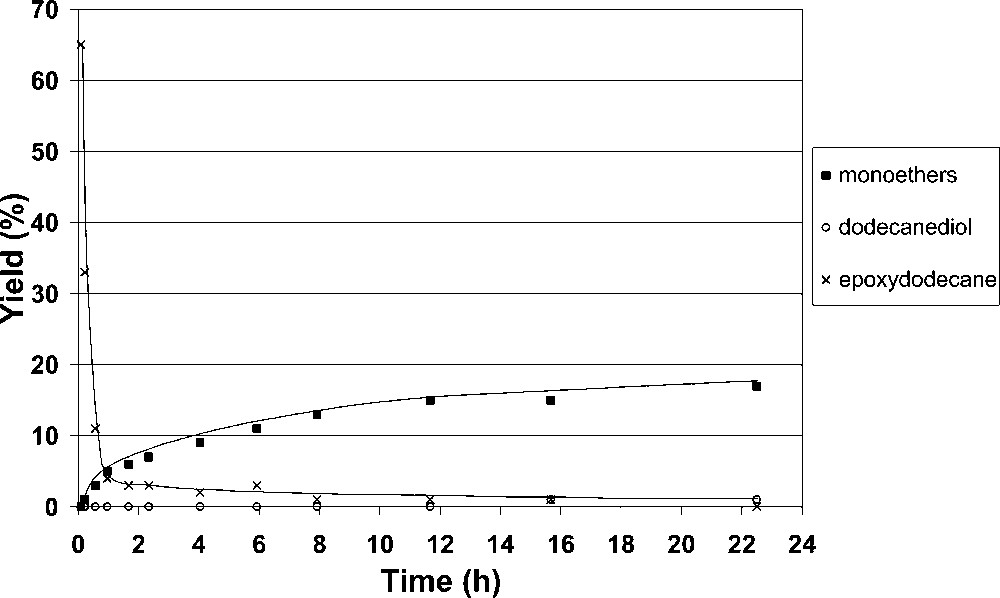
Sucrose etherification at 90 °C with 2 equiv of DABCO.
In order to check the previous hypothesis, the synthesis of the basic quaternary ammonium salt was carried out starting from an equimolar mixture of DABCO and 1,2-epoxydodecane heated at 110 °C. In DMSO, the reaction slowly proceeded whereas addition of water (30% w/w), rapidly led to the total consumption of the 1,2-epoxydodecane. NMR and mass spectroscopy clearly evidenced the formation of the quaternary ammonium salt, along with 23% of dodecanediol. During the reaction, an increase of the ‘crude pH’ was observed and, as illustrated in Table 1, this pH variation depends on the base nucleophilicity (Table 1). It is now clear that in the presence of a proton donor such as sucrose or water [37,38], a strongly basic quaternary ammonium salt was formed and this latter was able to deprotonate the sucrose, leading to the sucroethers formation.
Alternatively, it can be proposed that the quaternary ammonium salt could act as an ‘epoxide carrier’ [39–41] and subsequent nucleophilic substitution of the ammonium group by sucrose could also lead to sucroethers formation. The reaction of sucrose with 1,2-epoxydodecane in the presence of an excess of DABCO (2 equiv vs epoxide) led to the fast epoxide consumption and the major formation of the quaternary ammonium salt. Then a slow formation of sucroethers was observed despite the absence of remaining epoxide in the mixture (Fig. 2).
Similarly, reaction of the quaternary ammonium salt, synthesized from N,N-dimethyldodecylamine and 1,2-epoxydodecane [42], with sucrose and an extra amount of amine at 110 °C in a diluted aqueous solution of sucrose (25% w/w) led to 88% of dodecanediol after 10 h of reaction, whereas in a concentrated aqueous solution of sucrose (80% w/w), 2% of sucroethers were detected after 6 h of reaction. These experiments showed that the quaternary ammonium salt formed in situ can act as an electrophilic centre and react either with water or with sucrose to afford respectively dodecanediol or sucroethers. However this reaction is slow and cannot account for the catalytic sucroethers formation.
The reaction was also studied using potassium hydroxide as basic catalyst, looking at the influence of the base amount, the temperature and the water content on the yield in monoethers, the hydrolysis rate, the reaction time and the crude coloration. A direct relationship between the initial rate of monoethers and the potassium hydroxide content was observed. On the other hand, dodecanediol formation increased, while the catalyst amount decreased.
Surprisingly, a base consumption was observed during the catalytic process and was clearly evidenced by carrying out the reaction with a lower amount of base (0.17 equiv instead of 0.28 equiv). The catalytic reaction stopped after 14 h, but immediately started again after addition of an extra amount of base (0,8 equiv) (Fig. 3).
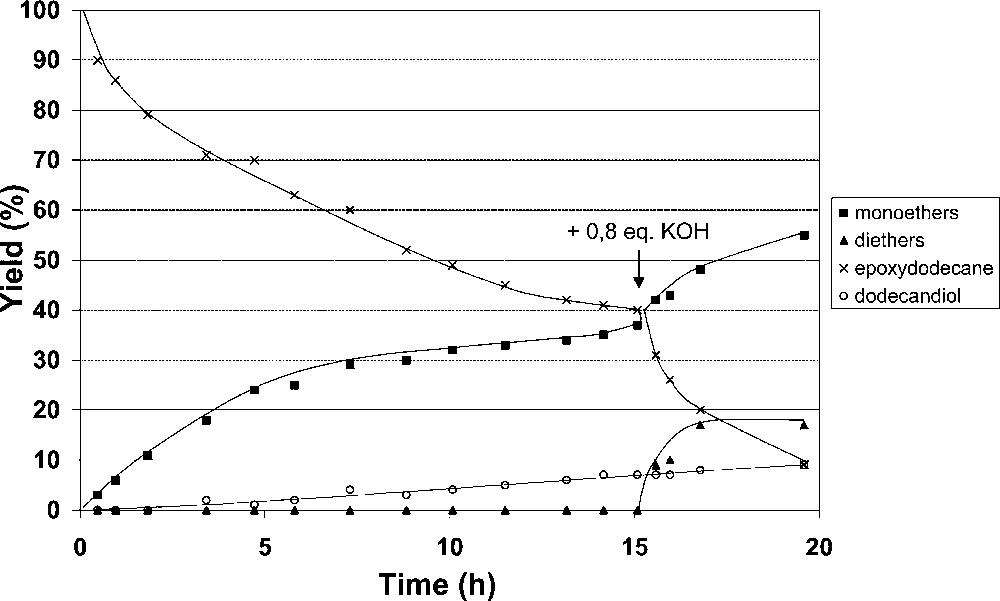
Addition of an extra amount of base during the reaction progress (95 °C).
Various tests aiming at understanding the base consumption were performed. At first, basic degradation of sucrose was incriminated, but the reaction carried out after pre-heating of potassium hydroxide in a mixture of epoxide and DMSO also evidenced a lower initial rate and a lower yield in monoethers. This showed that thermal degradation in basic medium of the epoxide or the solvent itself was also contributing to the base consumption.
Decreasing the temperature from 110 to 90 °C normally led to a slower reaction rate (14 h instead of 8 h) but with a lower coloration (light brown instead of dark brown), less dodecanediol formation and unchanged yields in sucroethers (Table 2).
The effect of water in the catalytic process was finally studied. Addition of up to 10% of water did not change the catalytic activity but water favoured the dissolution of the base and strongly decreased the medium coloration.
4 Heterogeneous catalysis
In order to easily recover and reuse the catalyst at the end of the reaction, we focussed our studies towards the use of heterogeneous catalysts. We previously showed that nucleophilic amines and hydroxide anions were able to catalyse the sucrose etherification. Based on these results, we investigated the ‘one-pot’ catalytic sucrose etherification over a weakly basic anion-exchange resin bearing dimethylamino groups (A21) and a strongly basic type-I anion-exchange resin functionalised with quaternary ammonium hydroxide group (A26) (Fig. 4).
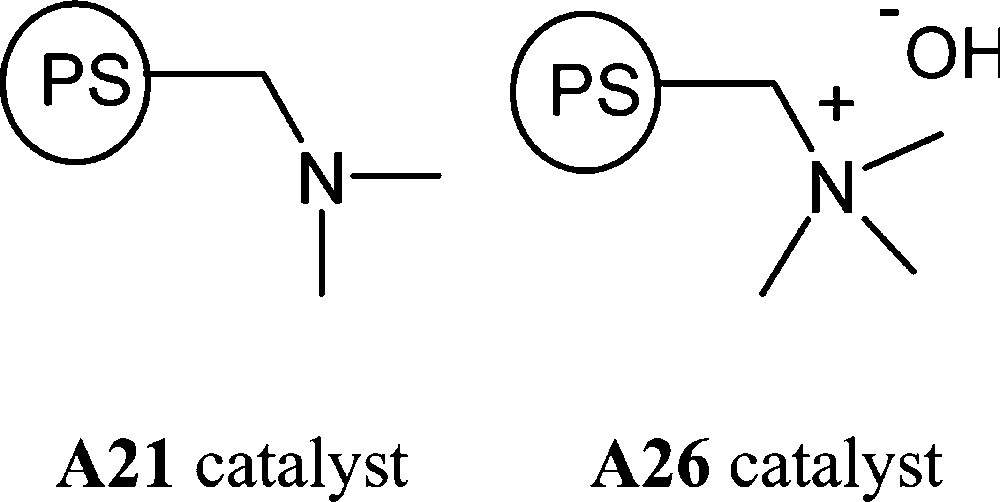
Basic insoluble polystyrene catalysts.
4.1 Reaction in dimethylsulfoxide
The catalytic etherification of sucrose was first performed at 110 °C in DMSO using a sucrose/epoxydodecane molar ratio of 4.
In the case of the A21 resin a very low catalytic activity was observed. After 20 h of reaction, only 24% of sucroethers were formed. In the same way, an important production of dodecanediol (45%) and a thermal epoxide degradation (31%) were observed. These major undesirable side products were mainly the result of the non-miscibility of the epoxydodecane in the sucrose phase.
In order to make easier the contact between the sucrose and the epoxydodecane, a phase transfer agent such as cetyltrimethylammonium bromide (CTAB) was added in the reaction mixture. As expected, the amount of side products was considerably decreased and sucroethers were formed in 80% yield (Table 3).
Catalytic etherification of sucrose over strongly basic anion-exchange resin
Catalyst | Solvent | SuOH/epoxide | T (°C) | Time (h) | Monoethers (%) | Diethers (%) | Dodecanediol (%) | Other (%) |
A26 resin | DMSO | 4 | 110 | 13 | 70 | 10 | 10 | 10a |
Me4NOH | DMSO | 4 | 110 | 1.5 | 79 | 14 | 2 | 5a |
A26 resin | DMSO | 1 | 110 | 13 | 41 | 24 | 17 | 18a |
Me4NOH | DMSO | 1 | 110 | 1 | 47 | 50 | 3 | 0a |
A26 resin | H2O/CTAB | 4 | 100 | 13 | 18 | 27 | 18 | 37a |
Me4NOH | H2O/CTAB | 4 | 100 | 6.5 | 13 | 15 | 27 | 45a |
a Products not detected by HPLC (polysucroethers and epoxid degradation products).
To avoid the use of CTAB without preventing the sucroether formation, we focussed our studies towards the use of a strongly basic type-I anion-exchange resin bearing quaternary ammonium hydroxide groups (A26). Using the same experimental conditions, but without adding CTAB, the A26 anion-exchange resin exhibited a higher catalytic activity than the A21 catalyst (Table 3). After 13 h of reaction, the 1,2-epoxydodecane was totally consumed and 80% of sucroethers were produced (Fig. 5).
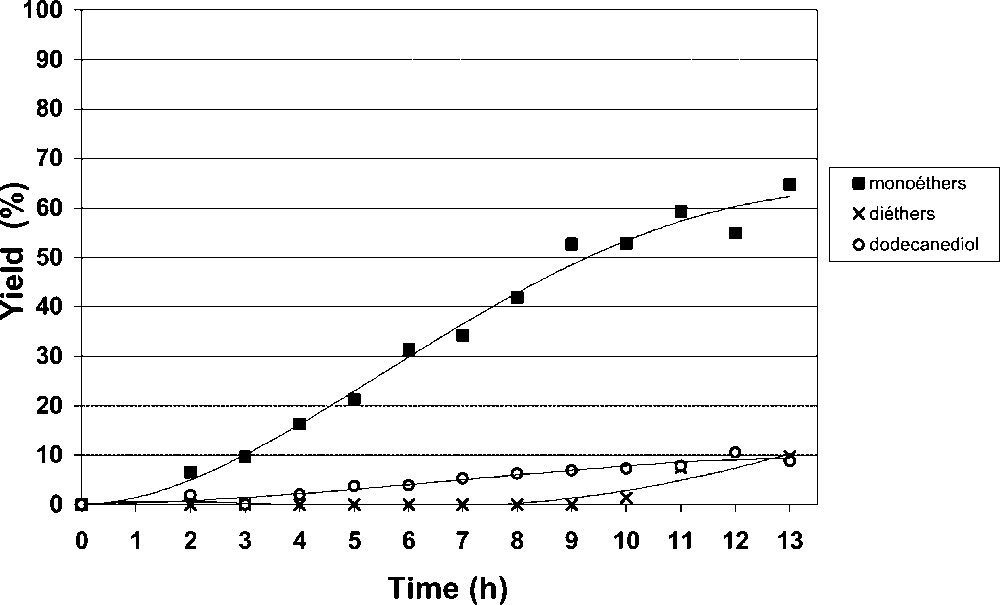
Catalytic etherification of sucrose over A26 resin.
4.1.1 Catalytic cycle
Acido-basic equilibriums are the main driving forces of the catalytic cycle. However, many basic species such as hydroxide, ammonium sucrate (I) or ammonium dodecanediolate (III) are formed during the catalytic process and each of them can be responsible for the catalytic activity (Fig. 6). In order to clarify the catalytic mechanism pathway, few cross-reaction tests were undertaken.
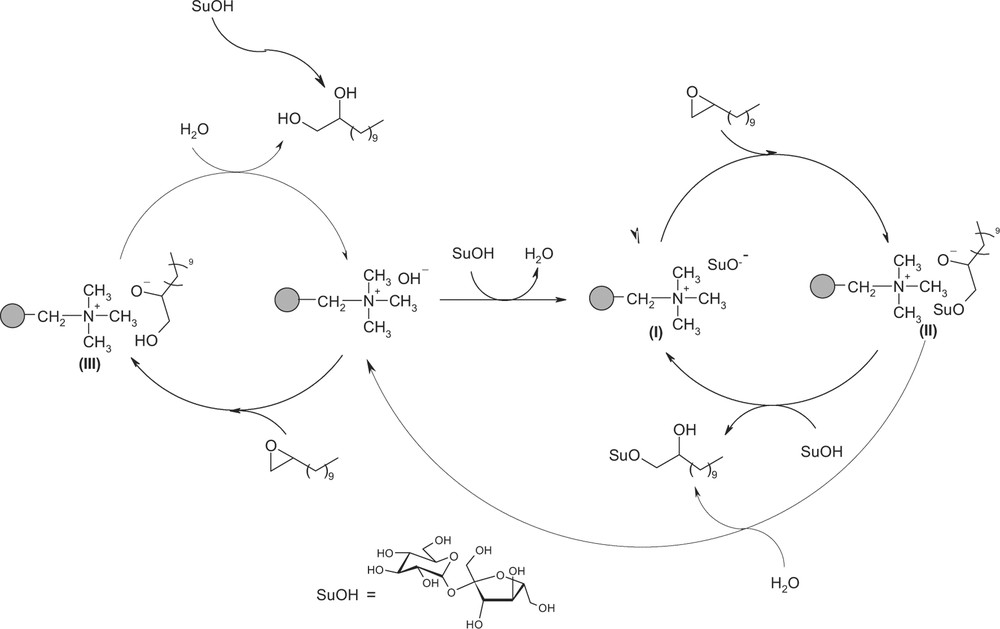
Catalytic mechanism pathway.
Sucrose was reacted with a catalytic amount of A26 resin and stirred in water to generate a supported quaternary ammonium sucrate (I). This modified resin (I) was recovered by filtration, thoroughly washed with warm anhydrous DMSO to remove the excess of sucrose and then used as catalyst.
After 23 h of reaction in DMSO, major epoxydodecane degradation occurred and only 10% of sucroethers were detected. This very low catalytic activity shows that the intermediate (I) does not easily react with the 1,2-epoxydodecane to form sucroethers. The highly hydrophilic catalytic sites of the modified resin (I) probably inhibit the 1,2-epoxydodecane adsorption, decreasing the catalytic reaction rate and increasing the thermal epoxide degradation.
When the same modified resin (I) was used in the presence of 10 mol% of dodecanediol, a higher catalytic activity was observed and sucroethers were formed in 80% yield. During the catalytic reaction, the dodecanediol probably acts as a phase transfer agent making easier the contact between the epoxydodecane and the grafted sucrate (I), allowing the sucroether formation.
In order to validate the dodecanediol role during the catalytic process, this latter was replaced by 10 mol% of CTAB. The use of the modified resin (I) with CTAB led to the same catalytic activity than that observed with dodecanediol, enhancing the phase transfer agent role played by the dodecanediol.
Interestingly, the same reaction can be carried out under dry conditions without notable change of the catalytic activity, indicating that water is not a key intermediate regarding the protonation of the catalytic species (II) and (III).
On the other hand, in the case of the A21 resin, all attempts to use the dodecanediol instead of the ionic CTAB were unsuccessful, probably because of its less effective surface activity.
We propose here a possible mechanism pathway to explain the catalytic activity observed with the A26 resin (Fig. 6). The first step is the deprotonation of the sucrose moiety by the hydroxide anion to form an ammonium sucrate (I). This latter can then react with the 1,2-epoxydodecane to form an ammonium sucroether alcoholate (II), which can be protonated by sucrose leading to the formation of sucrose hydroxyalkylether and regeneration of the catalyst (I).
This reaction is initiated by the side production of dodecanediol, which acts as a phase-transfer agent and allows the reaction to start. The dodecanediol is formed by a basic hydrolysis of the epoxydodecane and, despite the use of a hydroxide anion, its production remains low compared to that of sucroethers.
4.1.2 Comparison with the homogeneous catalysis
Prior to compare our results with those of homogeneous catalysts, it was necessary to estimate the amount of basic sites active during the heterogeneous catalytic process. One of the possible methods consisted in reacting sucrose with a catalytic amount of A26 resin in water. The catalyst was then filtered off, the water evaporated and the unreacted sucrose was weighted. Only 0.75 g of sucrose reacted with 0.6 g of catalyst, indicating that only 3.67 mmol OH–/g are active during the catalytic process, while the A26 anion-exchange capacity given by Rohm and Hass is higher (4.40 mmol OH–/g). However, this latter anion-exchange capacity was calculated for a hydroxide exchange with a continuous flow of a chloride solution. We suggest here that the sucrose moiety, much more hindered than the chloride anion, does not access to all grafted hydroxide groups as easily as the chloride anion.
Based on this result, all comparative studies were done with the appropriate amount of homogeneous catalyst (tetramethylammonium hydroxide) (Table 3).
Using a sucrose/epoxydodecane molar ratio of 4, the catalytic reaction was almost 10 times faster with the homogeneous catalyst (1.5 h) than with the A26 resin (13 h). This difference is explained by the important steric hindrance of the solid catalyst. In both cases, no major difference of yield and selectivity was observed. However, the amount of side products was less important under homogeneous conditions (8% vs 20%) and results from a shortest reaction time (Table 3).
When the ratio sucrose/epoxydodecane decreased from 4 to 1, an expected change of the selectivity was observed with an increase of the diether amounts (Table 3). Surprisingly, with the tetramethylammonium hydroxide, an equimolar production of mono and diethers was obtained, whereas the A26 resin mainly afforded monoethers (Table 3). As previously mentioned, the higher steric hindrance of the heterogeneous catalytic surface prevents the access of sucrose monoethers to all grafted catalytic sites as easily as in the case of the homogeneous catalyst. Consequently, diethers are more rapidly formed with the homogeneous catalyst inducing a difference of selectivity.
The monoether regioisomer distribution [15] was rather similar between the A26 resin and the tetramethylammonium hydroxide (reactive positions: 2(34%) > 1′(23%) > 3′12%) > 6,6′,4,4′(31%)), indicating that sucrose reacts with the catalytic sites in a similar way, either at the resin surface or with the homogeneous catalyst.
4.2 Reaction in water
When the catalytic reaction was carried out in water either with the homogeneous catalyst or the A26 resin, sucrose was not converted to sucroethers. The very poor affinity of the 1,2-epoxydodecane for the aqueous phase prevents the dodecanediol formation and the catalytic reaction does not take place. As described for the A21 resin, addition of 10 mol% of CTAB starts immediately the reaction.
The use of water as solvent led to a dramatic change of selectivity (Table 3). TLC analysis revealed that sucrose polyethers (not detected by HPLC) were present in a large amount showing that water favours the polyetherification of sucrose, as it was already observed in the literature for the sucroester synthesis [43]. This result shows that solvent plays a very important role but up to now we are not able to discriminate between different parameters to explain this surprising change of selectivity.
4.3 Stability of the resin
At the end of the reaction, the A26 resin was easily removed by filtration and reused. A decrease of the catalytic activity was observed since only 25% of sucroethers were detected during the second run. This loss of reactivity is due to the resin degradation at 110 °C. Both polystyrene crosslinked with a few percent of divinylbenzene [24] and quaternary ammonium hydroxides are known to be unstable at high temperature [44–46]. Physical characteristics provided by Rohm and Hass indicate that the A26 resin is stable up to 60 °C. In order to check the resin stability under our experimental conditions, the resin was heated at 110 °C for 13 h prior to use. After filtration, the resin was used as catalyst and only 20% of sucroethers were detected, which confirmed the poor thermal stability of the A26 resin in DMSO. At the same time, to ensure that no catalytic sites leaching occurred during the catalytic process, the reaction was also performed with the previous heated resin filtrate. No sucroethers were detected, indicating that no leaching occurred during the reaction.
5 Conclusion
Tertiary amines react with 1,2-epoxydodecane to generate a strongly basic quaternary ammonium alcoholate intermediate, which is able to deprotonate the sucrose and catalyse the reaction. The presence of a small proportion of water does not change the sucroether yields, but an easier dissolution of the base in the epoxide phase.
A strongly basic quaternary ammonium type-I anion-exchange resin (OH– form) A26 catalyses the sucrose etherification at low temperature and exhibits the same catalytic activity than the homogeneous catalyst. Compared to the tetramethylammonium hydroxide, the A26 resin is slightly more selective and more easily separated from the reaction products, leading to a cleaner catalytic process.
However, due to the poor thermal stability of the A26 resin, we are now working on the grafting of strongly basic sites on different solid supports chemically more robust than a resin.
Acknowledgements
Authors are grateful to Beghin-Say, CNRS and to the AGRICE action (contract No. 01 01 016) for their financial supports. IA particularly thanks ADEME and the ‘Région Poitou-Charentes’ (France) for their PhD grant.